1
Learning Goals
-
Explain how natural selection can result in adaptive evolution
-
Identify sources of genetic variations in populations
-
Define adaptation as it relates to natural selection
-
Distinguish between convergent and divergent evolution
Introduction
Darwin, Wallace and Natural Selection
In the mid-nineteenth century, two naturalists, Charles Darwin and Alfred Russel Wallace, independently conceived and described the actual mechanism for evolution. On the ship H.M.S. Beagle, Darwin traveled around the world, including stops in South America, Australia, and the southern tip of Africa. Darwin’s journey, like Wallace’s later journeys to the Malay Archipelago, included stops at several island chains. Darwin’s last being the Galápagos Islands west of Ecuador. On these islands, Darwin observed species of organisms on different islands that were clearly similar, yet had distinct differences.
For example, the ground finches inhabiting the Galápagos Islands comprised several species with a unique beak shape (Fig. 1). What Darwin noted was that the species on the islands had a graded series of beak sizes (small to large) and shapes (width and depth). In addition, between the most similar species, there were very small differences. Darwin also observed that these finches closely resembled another finch species on the South American mainland. As a result of his observations, Darwin hypothesized that the island species might be the result of modifications of one original mainland species. Upon further study, he realized that each finch’s varied beaks helped the birds acquire a specific type of food. For example, seed-eating finches had stronger, thicker beaks that helped break seeds. Insect-eating finches had spear-like beaks, allowing them to stab their prey.
Over their travels, Wallace and Darwin both observed similar patterns to those seen in the finches in other organisms. Based on their observations they each independently developed the same explanation (natural selection) for how and why such changes could take place. Natural selection, or “survival of the fittest” is the increased reproductive success of individuals with traits that enhance survival and reproduction. This differential reproduction in individuals within a population can lead to evolutionary change.
Natural Selection
Variation in Populations
Genetic diversity in a population comes from two main mechanisms: mutation and sexual reproduction. Mutation, a change in DNA, is the ultimate source of new alleles, or new genetic variation in any population. The genetic changes that mutation causes can have one of three outcomes on the phenotype.
(1) A mutation affects the organism’s phenotype in a way that gives it reduced fitness—the lower likelihood of survival or fewer offspring.
(2) A mutation may produce a phenotype with a beneficial effect on fitness.
(3) A mutation may also have no effect on the phenotype or the organism’s fitness.
Most mutations do not affect the phenotype of the organism. Sexual reproduction can also lead to changes genetic diversity: when two parents reproduce, unique combinations of alleles assemble to produce the unique genotypes and thus phenotypes in each offspring.
Adaptations
Divergent and Convergent Processes
We can see such divergent evolution in the forms of the reproductive organs of flowering plants. The flowers share the same basic anatomies that may include sepals, petals, pistils, and stamen. However, the individual shapes of the structures can result in very different appearances. This is often the result of selection in different physical environments and adaptations to different kinds of pollinators.
In other cases, similar phenotypes evolve independently in distantly related species. For example, flight has evolved in both bats and insects, and they both have structures we refer to as wings, which are adaptations to flight. However, bat and insect wings evolved from very different original structures.
The white feathers of arctic birds and the white fur of arctic mammals are other examples of convergent evolution. These similarities occur not because of common ancestry, but because of similar selection pressures. These physical changes occur over enormous time spans and help explain how evolution occurs. Natural selection acts on individual organisms, which can then shape an entire species. Although natural selection may work in a single generation on an individual, it can take thousands or even millions of years for an entire species’ genotype to evolve. It is over these large time spans that life on earth has changed and continues to change.
Summary
End of Section Review Questions:
Review: Evolutionary Patterns
2) __________evolution occurs when organisms that are NOT closely related become more similar over long periods of time.
Review: Components of Natural Selection
Review: Addressing a common misconception
4) Explain why it is populations that become more adapted to environments and not individuals.
References
Modification of OpenStax Biology 2nd Edition, Biology 2e. OpenStax CNX. Nov 26, 2018 18.1 Understanding Evolution. https://cnx.org/contents/jVCgr5SL@15.1:zE5eDZcY@10/18-1-Understanding-Evolution
Image Attribution
Figure 1. Image by John Gould (14.Sep.1804 – 3.Feb.1881) [Public domain], via Wikimedia Commons
Figure 2. Image courtesy of Debivort [GFDL (http://www.gnu.org/copyle“/fdl.html) CC-BY-SA-3.0
Figure 3. Left image courtesy of Rick Hansen,U.S. Fish and Wildlife Service. Public domain. Right image courtesy of Richard Bartz, Munich aka Makro Freak CC BY-SA 2.5
Figure 4. Left image courtesy of IronChris [GFDL (http://www.gnu.org/copyle“/fdl.html), CC-BY-SA-3.0 . Right image courtesy of CNX OpenStax CC BY 4.0, modified by D. Jennings (Jan 2019).
Learning Goals
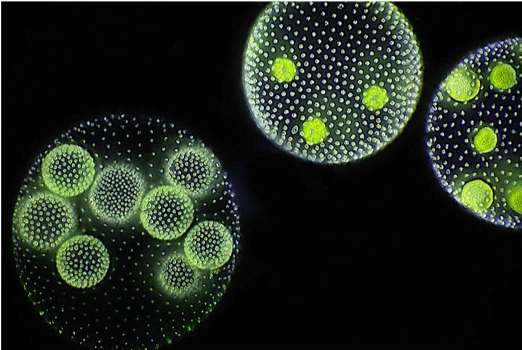
By the end of this reading you should be able to:
- Explain the advantages of simple multicellularity
- Describe the evidence that supports the evolution of simple multicellularity in some protists
- Discuss the needed adaptations for simple multicellularity
Introduction
Among the Bacteria and Archaea, each cell usually functions as an individual, growing and reproducing, moving from one place to another, taking in nutrients, and both sensing and transmitting molecular signals. In contrast, multicellular organisms contain millions (or more) cells that work in close coordination. In your own body, for example, different cells are specialized for specific functions, so that while your body as a whole can perform the broad range of tasks accomplished by microorganisms, individual cells, for the most part, cannot. Cells lining the intestine absorb food molecules, but nutrients must then be transported to other parts of the body. Lungs take up oxygen from inhaled air, but this, too, must be distributed to other tissues and organs. And while cells at the body's surface sense signals from the environment, the signals affecting interior cells come mostly from surrounding cells. While the biological gulf between microbes and multicellular organisms is enormous, multicellularity has evolved a half dozen times in different groups.
The phylogeny of multicellularity
Most prokaryotic organisms are composed of a single cell, although some form simple filaments or live in colonies. A few types of bacterium, notably some cyanobacteria, differentiate to form several distinct cell types. No bacteria, however, develop macroscopic bodies with functionally differentiated tissues. Only eukaryotes have evolved those. Simple multicellular organisms, composed of multiple similar cells, occur widely within the eukaryotic tree of life, and a few of these evolved into more complex multicellular organisms characterized by differentiated tissues and organs. How are these different types of organization distribute on the eukaryotic tree of life?
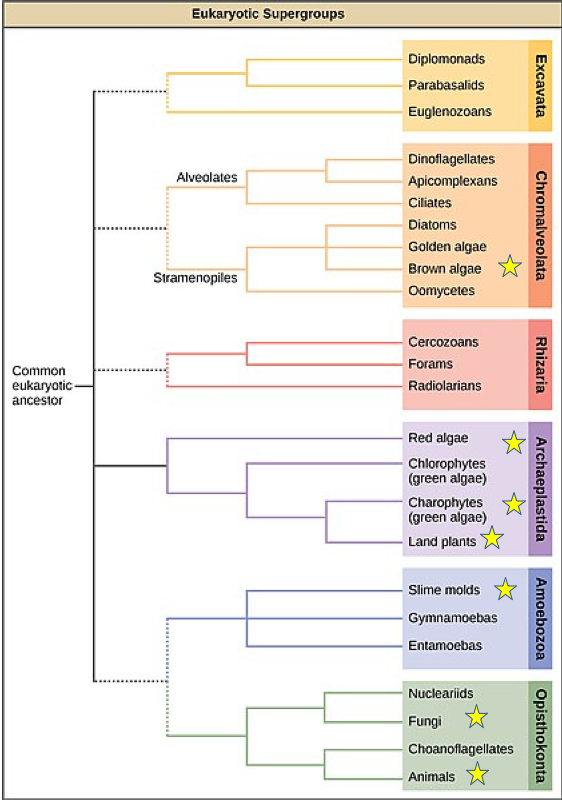
A recent survey of eukaryotic organisms recognized 119 major groups within the supergroups. Of these, 83 contain only single-celled organisms, predominantly cells that engulf other microorganisms or ingest small organic particles, photosynthetic cells that live suspended in the water column, or parasitic cells that live within other organisms. Each of the 36 remaining branches exhibits some cases of simple multicellularity, mostly in the form of filaments, hollow balls, or sheets of little-differentiated cells. More complex multicellularity has evolved only in six eukaryotic groups: animals, fungi, brown algae, red algae, green algae, and land plants.
Thinking Question:
Advantages of simple multicellularity
What selection did pressures favor the evolution of simple multicellular organisms from single-celled ancestors? One selective advantage is that multicellularity helps organisms avoid getting eaten. A number of experiments using single-celled green algae and exposure to a single cell predator have demonstrated the selection pressure for multicellularity. In the presence of the predators, within 10 to 20 generations, most of the algae were living in eight-cell colonies that were essentially invulnerable to predator attacks due to their larger size.1,2
Another advantage is that multicellular organisms may be able to maintain their position on a surface or in the water column better than their single-celled relatives. Seaweeds, for example, live anchored to the seafloor in places where light and nutrients support growth. Scientists have studied these phenomena using both single-celled algae and yeast cultures.3,4 When exposed to consistent shaking in culture, the number of cells that formed aggregates (clumps) increases over a number of generations. By clumping together the cell groups settled on the bottom of the cultures and thus were exposed to less vigorous conditions.
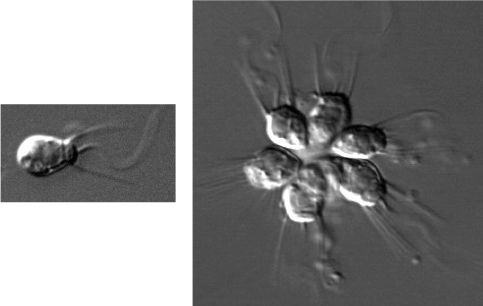
Feeding provides a third potential advantage: In colonial heterotrophs such as the stalked ciliate Epistylis, the coordinated beating of flagella assists feeding by directing currents of food-laden water toward the cells. Studies using the choanoflagellate Salpingoeca rosetta, demonstrated a link between the presence of the prey species and the development of multicellular colonies (formation of a rosette). These colonies, unlike the ones in the yeast and algae mentioned earlier, appear to the result of cell division rather than cell aggregation.5
Adaptations need for multicellularity
The genome of the choanoflagellate Monosiga brevicollis provides a fascinating insight into the evolution of cell adhesion molecules. Choanoflagellates, the closest protistan relatives of animals, are unicellular microorganisms. Therefore, it came as a surprise that the genes of M. brevicollis code for many of the same protein families that promote cell adhesion in animals. This includes the genes for both cadherin and integrin proteins which in animals are involved in supporting epithelia. Clearly, these proteins are not supporting epithelia in M. brevicollis, so what are they doing?
One approach to an answer came from the observation that cells stick not only to one another but also to rock or sediment surfaces. Proteins that originally evolved to promote adhesion of individual cells to sand grains or a rock surface may have been modified during evolution for cell-cell adhesion. It is also possible that adhesion molecules were originally used in the capture of bacterial cells, to make prey adhere to the predator.
To date, cadherins have been found only in choanoflagellates and animals, but proteins of the integrin complex extend even deeper into eukaryotic phylogeny-they are found in single celled protists that branch near the base of the opisthokont superkingdom. Such a phylogenetic distribution provides strong support for the general hypothesis that cell adhesion in animals resulted from the redeployment of protein families that evolved to perform other functions before animals diverged from their closest protistan relatives.
Thinking Question:
Adhesion but no differentiation
In many protists, cell adhesion molecules cause adjacent cells to stick together, but there are few specialized cell types and relatively little communication or transfer of resources between cells. Most or all of the cells in simple multicellular organisms retain a full range of functions, including reproduction. As a result the organism/colony usually pays only a small penalty for individual cell death. Importantly, in simple multicellular organisms, nearly every cell is in direct contact with the external environment, at least during phases of the life cycle when the cells must acquire nutrients, which means that each can take up nutrients and excrete wastes directly.
Simple multicellularity occurs most prominently among algae, although stalk-like colonies of particle feeders have evolved in at least three groups of heterotrophic protists. In addition, simple filamentous fungi exist that can absorb organic molecules as sources of carbon and energy. Four eukaryotic groups have achieved temporary simple multicellularity by a different route, aggregating during just one stage of the life cycle. Slime molds are the best-known example.
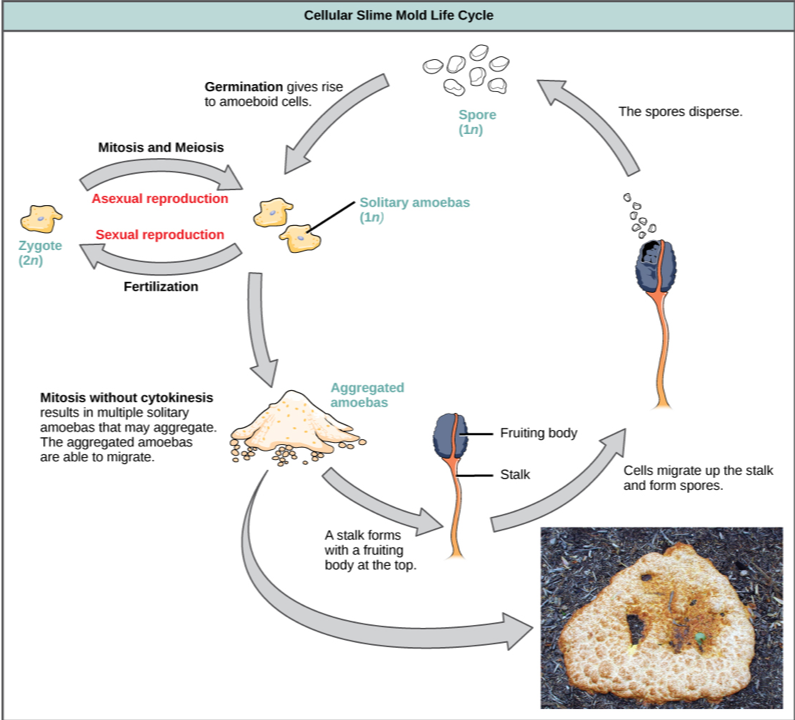
Six groups (two algal, three protozoan, and one fungal) include species characterized by coenocytic organization. In coenocytic organisms, the nucleus divides multiple times, but the cell does not, so the nuclei are not partitioned into individual cells. The result is a large cell-sometimes even visible to the naked eye with many nuclei. The green algae Codium and Caulerpa, found along the shorelines of temperate and tropical seas, respectively, are common examples of coenocytic organisms. In addition, acellular slime molds are also coenocytic and can be so large that they look like fungi to us whereas they are only one really really large cell. There is no evidence that any coenocytic organisms evolved from truly multicellular ancestors, nor have any given rise to complex multicellular descendants.
Review Question:
Summary
Given the benefits associated with being multicellular, it is no surprise that multiple lineages independently evolved multicellularity over time. This likely took advantage of traits that were already present in the organisms to help those ancestral cells adhere to a substrate. The first multicellular organisms were likely simple and the cells merely stuck together but did not differentiate and have different functions for the whole. Other lineages evolved processes in which the nucleus divides multiple times in the same cell, forming a coenocytic cell, but there is no evidence that multicellularity evolved from coenocytic cells.
End of Section Review Questions:
2) What do features would you expect SIMPLE multicellular organisms have in common?
References
1 Boraas, M. E., D. B. Seale, and J. E. Boxhorn. 1998. Phagotrophy by a flagellate selects for colonial prey: a possible origin of multicellularity. Evol. Ecol. 12:153–164 https://link.springer.com/article/10.1023/A:1006527528063
2 Matthew D. Herron, Josh M. Borin, Jacob C. Boswell, Jillian Walker, I-Chen Kimberly Chen, Charles A. Knox, Margrethe Boyd, Frank Rosenzweig, William C. Ratcliff. 2018. De novo origin of multicellularity in response to predation bioRxiv 247361; doi: https://doi.org/10.1101/247361
3 Ratcliff, W. C., Denison, R. F., Borrello, M. & Travisano, M. Proc. Natl Acad. Sci. USA advance online publication http://dx.doi.org/10.1073/pnas.1115323109 (2012).
4 Ratcliff, William C., et al. "Experimental evolution of an alternating uni-and multicellular life cycle in Chlamydomonas reinhardtii." Nature communications 4 (2013): 2742. https://www.nature.com/articles/ncomms3742
5 Fairclough SR, Dayel MJ, King N. Multicellular development in a choanoflagellate. Curr Biol. 2010;20(20):R875-6. doi: 10.1016/j.cub.2010.09.014
Image attribution
Figure 1. Image courtesy of Frank Fox [CC BY-SA 3.0 de (https://creativecommons.org/licenses/by-sa/3.0/de/deed.en)], via Wikimedia Commons
Figure 2. Image courtesy of CNX OpenStax [CC BY 4.0 (https://creativecommons.org/licenses/by/4.0)], via Wikimedia Commons
Figure 3. Image courtesy of Mark J. Dayel [CC BY-SA 3.0 (https://creativecommons.org/licenses/by-sa/3.0)], via Wikimedia Commons
Figure 4. Image courtesy of CNX OpenStax [CC BY 4.0 (https://creativecommons.org/licenses/by/4.0)], via Wikimedia Commons
Learning Goals
By the end of this reading you should be able to:
- Describe the shared features of complex multicellular organisms
- Compare and contrast simple vs. complex multicellular organisms
- Explain the limitations to diffusion and the role of bulk flow in complex multicellular organisms
- Discuss the means by which cell adhesion, cell communication, and cell differentiation are accomplished in complex multicellular organisms
- Predict the order of acquisition of multicellular traits and the evolutionary consequences of complex multicellularity
Introduction
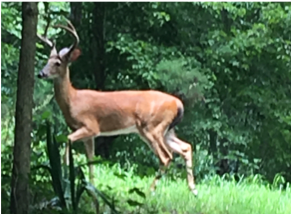
There are costs associated with multicellularity, particularly for complex multicellular organisms with differentiated reproductive tissues. In these organisms, most cells do not reproduce, instead of supporting the few that do. This requires cooperation among cells, but it creates opportunities for cells to "cheat"- to use nutrients for their own proliferation rather than the growth and reproduction of the organism as a whole. Complex multicellularity evolved at least six separate times in different eukaryotic groups; once in animal lineages, once in the green algal lineage that gave rise to land plants, twice in the fungi, once in the red algae, and once in the brown algae, producing the giant kelps that form forests in the sea.
Shared Features
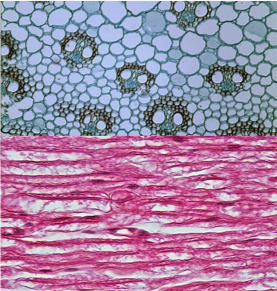
Complex multicellular organisms differ from one another in many ways, but they share three general features. (1) They have highly developed molecular mechanisms for adhesion between cells. (2) They display specialized structures that allow cells to communicate with one another. (3) They display complex patterns of cellular and tissue differentiation, guided by networks of regulatory genes. Without these features, complex multicellularity would be impossible.
For example, plants and animals both have differentiated cells and tissues with specialized functions. In plants, only some tissues photosynthesize or absorb organic molecules; other tissues transport food and oxygen through the body, and still, others generate the molecular signals that govern development. In both plants and animals, only a small subset of all cells contributes to reproduction. Because of this functional differentiation, cell or tissue loss can be lethal for the entire organism.
There is one more feature of complex multicellular organisms that is key to understanding their biology: They have a three dimensional organization, so only some cells are in direct contact with the environment. Cells that are buried within tissues, relatively far from the exterior of the organism, do not have direct access to nutrients or oxygen. Therefore, interior cells cannot grow as fast as surface cells unless there is a way to transfer resources from one part of the body to another. Similarly, interior cells do not receive signals directly from the environment, even though all cells must be able to respond to environmental signals if the organism is to grow, reproduce, and survive. Complex multicellular organisms, therefore, require mechanisms for transferring environmental signals received by cells at the body's surface to interior cells, where genes will be activated or repressed in response. Thus growth and development in complex multicellular organisms can be defined as increasing or decreasing gene expression in response to molecular signals from surrounding cells.
Review Question:
What are the characteristics that are found in both simple colonies of cells and complex multicellular organisms?
A) the ability of cells to communicate
Diffusion and Bulk Flow
A key functional challenge of complex multicellularity is transporting food, oxygen, and molecular signals rapidly across large distances within the body. How does oxygen get from the air in your lungs into your bloodstream? How does atmospheric carbon dioxide get into leaves? How does ammonia get from seawater into the cells of seaweeds? The answer to all three questions is the same: by diffusion. But oxygen absorbed by your lungs doesn't reach your toes by diffusion alone-it is transported actively, and in bulk, by blood pumped through your circulatory system. Water does not get from the roots to the leaves of plants by diffusion either, there are mechanisms at work to move it actively as well. For complex multicellular organisms to function, bulk flow of oxygen, nutrients, and signaling molecules, at rates and across distances far larger than can be achieved by diffusion alone is needed.
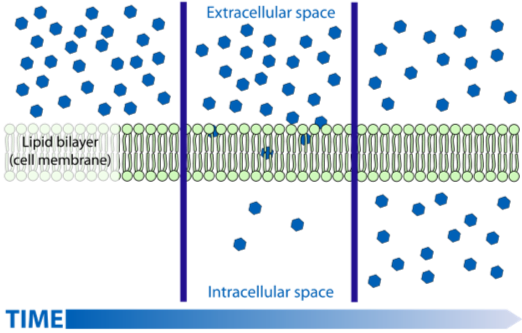
So why is diffusion effective only over short distances? Diffusion is the random motion of molecules, with net movement from areas of higher to lower concentration. The distance and speed at which molecules can diffuse depends on several factors: (1) how great the concentration difference is, large larger the differences the faster the diffusion (2) distance between concentration differences, the closer the two concentrations the faster the rate of diffusion (3) the size of the molecule, smaller molecules tend to diffuse faster than larger ones, and (4) the nature of the medium the molecule is diffusing in; molecules diffuse faster if the medium is less thick (think air vs. water). Because diffusion supplies key molecules for metabolism, it exerts a strong constraint on the size, shape, and function of cells and ultimately how eukaryotic organisms function.
Let's use oxygen as an example. Most eukaryotes require oxygen for respiration. If the cells within a tissue must rely on diffusion for their individual oxygen supply, the thickness of the tissue is limited by the concentration difference in oxygen between the cells in the tissue and the surrounding environment. The concentration difference between the two areas depends on the amount of oxygen in the environment and the rate at which oxygen is used for respiration inside the organism. The distance between the environment and the cell that is using oxygen also plays a role.
Review Question:
Which of these would increase the rate of diffusion of a molecule?
A) a high concentration gradient between two areas involved
Organisms can achieve larger sizes by circumventing limits imposed by diffusion.
Sponges can reach overall dimensions of a meter or more, but they actually consist of only a few types of cells that line a dense network of pores and canals and so remain in close contact with circulating seawater. The large size of a sponge is therefore achieved without placing metabolically active cells at any great distance from their environment. Similarly, in jellyfish, active metabolism is confined to thin tissues that line the inner and outer surfaces of the body. Essentially, a large flat surface is folded up to produce a three-dimensional structure. An increase in the surface area of a cell and or structure increases the area that is capable of interacting with the environment and ultimately the number of needed molecules to which a cell or structure has access.
Structures specialized for bulk flow
Bulk flow is any means by which molecules move through organisms at rates beyond those possible by diffusion across a concentration gradient. In humans and other vertebrate animals, the active pumping of blood through blood vessels supplies oxygen to tissues that may be more than a meter distance from the lungs. Our lungs gather the oxygen we need for respiration, but the lung is a prime example of diffusion in action, not a means of avoiding it. Because lung tissues have a very high ratio of surface area to volume, oxygen can diffuse efficiently from the air you breathe into lung tissue. A great deal of oxygen can be taken in this way, but how does it get from the lungs to our brains or toes? The distances are far too large for diffusion to be effective.
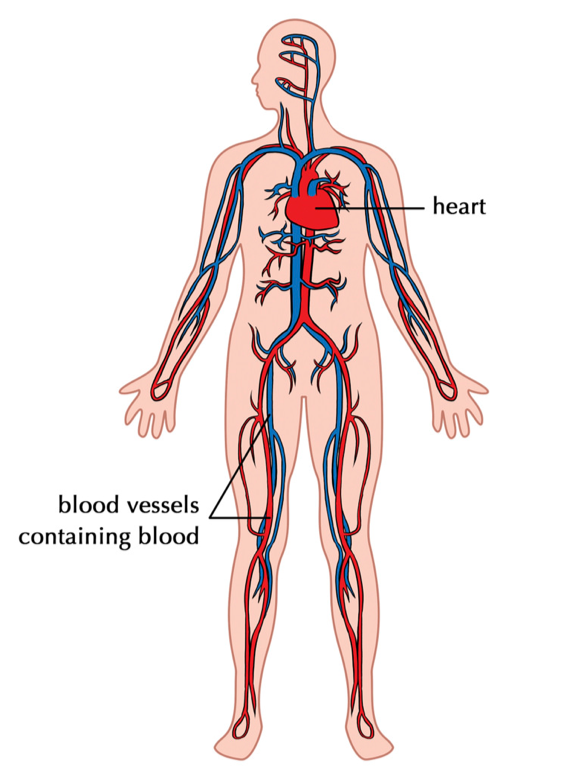
The answer is that oxygen binds to molecules of hemoglobin in red blood cells and then is carried through the bloodstream to distant sites of respiration. We circumvent diffusion by actively pumping oxygen-rich blood through our bodies. Most invertebrate animals lack well-defined blood vessels instead they have other mechanisms that circulate fluids freely throughout the body cavity. Indeed, without a mechanism like bulk flow, animals could not have achieved the range of size, shape, and function familiar to us.
Complex organisms other than animals also rely on bulk flow. A redwood tree must transport water upward from its roots to leaves that may be 100 m above the soil. If plants relied on diffusion to transport water, they would be only a few millimeters tall. How, then, do they move water? Plants move water by bulk flow through a system of specialized tissues powered by the evaporation of water from leaf surfaces. Vascular plants also have specialized tissues for the transport of nutrients and signaling molecules upward and downward through roots, stems, and leaves.
Multicellular fungi transport nutrients through networks of filaments that may be meters long, relying on osmosis to pump materials from sites of absorption to sites of metabolism. The giant kelps have an internal network of tubular cells that transports molecules through a body that can be tens of meters long. In general, when some cells within an organism are buried within tissues, far from the external environment, bulk flow is required to supply those cells with molecules needed for metabolism.
Other requirements for complex multicellular life
In addition to a means to move needed molecules over long distances and in sufficient concentration, there are three other general requirements for complex multicellular life: (1)Cells must stick together; (2) they must communicate with one another, and (3) they must participate in a network of genetic interactions that regulates cell division and differentiation. Once these are in place, the stage is set for the evolution of specialized tissues and organs in complex multicellular organisms.
Adhesion between cells
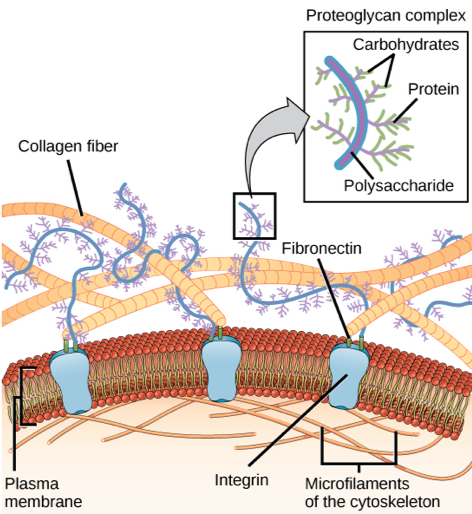
If a fertilized egg is to develop into a complex multicellular organism, it must divide many times, and the cells produced from those divisions must stick together. In addition, they must retain a specific spatial relationship with one another in order for the developing organism to function. Most animal cells release materials into the extracellular space. The primary components of these materials are proteins, and the most abundant protein is collagen. Collagen fibers are interwoven with carbohydrate-containing protein molecules called proteoglycans. Some cells attach themselves to this matrix by means of other transmembrane proteins called integrins and transmembrane proteins, especially cadherins, can also be involved in the formation of molecular attachments between cells. The extracellular matrix also allows the cells within the tissue to communicate with each other.
In plants, the cell wall connects cells together into tissues. The plant cell wall is composed predominately of cellulose fibers which are embedded in a matrix of pectin and hemicellulose. These molecules allow plant cells to attach to each other to create the complex tissue structures that support the overall plant structure. Fungi also have cell walls, although they are composed of chitin rather than cellulose, that are involved in connecting the cells together into multicellular structures.
Communication between cells
In complex multicellular organisms, it is not sufficient for cells to adhere to one another. They must also be able to communicate. Communication is important during development, guiding the patterns of gene expression that differentiate cells, tissues, and organs. The functional integration of cells within tissues and tissues within organs also depends on the flow of information among cells. Cells communicate using signaling molecules (generally a protein) synthesized by one cell which binds with a receptor protein on the surface of a second cell. This interaction can essentially flip a molecular switch that activates or represses gene expression in the receptor cell's nucleus or stimulates another response from the receiving cells. Molecular evidence indicates that many of the signaling pathways used for communication between cells in complex multicellular organisms first evolved in single-celled eukaryotes.
Thinking Question:
What function might molecular signals and receptors have had in the ancestors of complex organisms?
All cells have transmembrane receptors that respond to signals from the environment. In some cases, the signal is a molecule released by a food organism (such as the bacteria that induce simple multicellularity in choanoflagellates), and in some cases the cells sense nutrients, temperature, or oxygen level. Single celled eukaryotes also communicate with other cells within the same species, for example, to ensure that two cells can find each other to fuse in sexual reproduction. Signaling between two cells within a complex multicellular organism (plant, animal, or fungal) can be seen as a variation on this more general theme of a cell responding to other cells and the physical environment.
While all eukaryotic cells have molecular mechanisms for communication between cells, complex multicellular organisms have distinct cellular pathways for the movement of molecules from one cell to another. For example, animals more complex than sponges have gap junctions, protein channels that allow ions and signaling molecules to move from one cell into another. Gap junctions not only help cells to communicate with their neighbors, they allow targeted communication between a cell and specific cells adjacent to it.
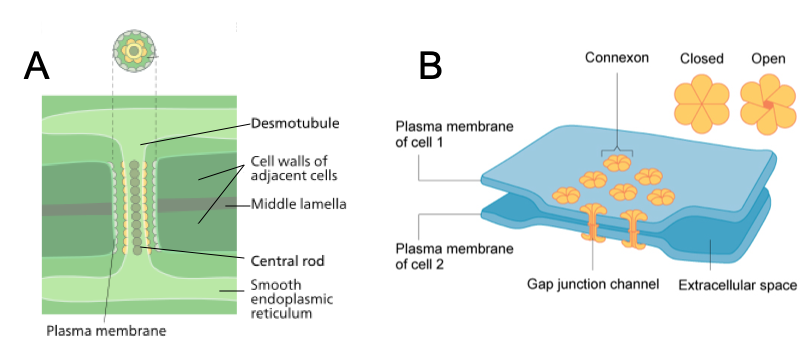
Plants, in contrast, have intercellular channels are lined by extensions of the cell membrane. Tubules running through these channels connect the endomembrane systems of the two cells. Like gap junctions, plasmodesmata permit signaling molecules to pass between cells in such a way that they can be targeted to only one or a few adjacent cells. Complex red and brown algae also have plasmodesmata, and complex fungi have pores between cells that enable communication by means of cytoplasmic flow. As similar channels do not occur in most other eukaryotic organisms, they appear to represent an important step in the evolution of complex multicellularity.
Review Question:
Genetic program for coordinated growth and cell differentiation
In multicellular organisms, growth and development is the result of molecular communication between cells. Cells have different fates depending on which genes are switched off or on, and genes are switched off and on by the molecular signals that cells receive. A signal commonly alters the production of proteins-inducing the reorganization of the cytoskeleton, for example. As a result, a stem cell may become an epithelial cell, or a muscle cell, or a neuron. This observation leads to another question: What causes the same gene to be turned on in one cell and off in another? The ultimate answer is that two cells in the same developing organism can be exposed to very different environments.
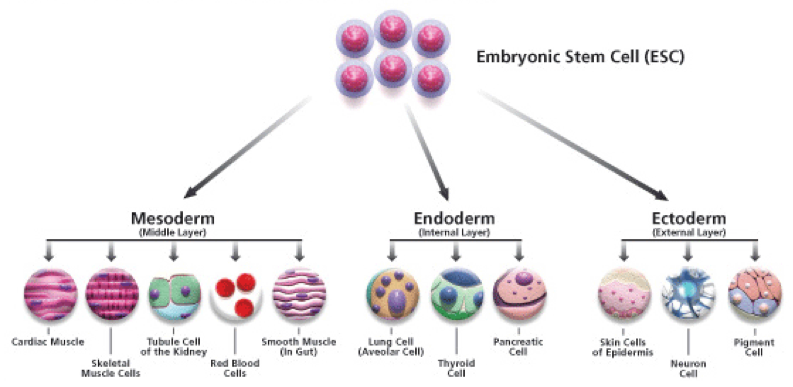
When we think about development as a process of programmed cell division and differentiation, the link to unicellular ancestors becomes clearer. Many biological innovations accompanied the evolution of complex multicellularity, but the differentiation of distinct cell types is not one of them. Many unicellular organisms have life cycles in which different cell types alternate in time, depending on environmental conditions. For example, if we experimentally starve dinoflagellate cells, two cells undergo sexual fusion to form morphologically and physiologically distinct resting cells protected by thick walls. That is, a nutrient shortage induces a change in gene expression that leads to the formation of resting cells. When food becomes available again, the cells undergo a meiotic cell division to form new feeding cells. Many other single-celled eukaryotes form resting cells in response to environmental cues, especially deprivation of nutrients or oxygen.
The innovation of complex multicellularity was to differentiate cells in space instead of time. In a three-dimensional multicellular organism, only surface cells are in direct contact with the outside environment. Interior cells are exposed to a different physical and chemical environment because nutrients, oxygen, and the light become less abundant with increasing depth within tissues. In effect, there is a gradient of environmental signals within multicellular organisms. We might, therefore, hypothesize that in the earliest organisms with three-dimensional multicellularity, a nutrient or oxygen gradient triggered oxygen- or nutrient-starved interior cells to differentiate, much as happens to trigger the formation of resting cells in their single-celled relatives. With increasing genetic control of cellular responses to signaling gradients, the seeds of complex development were sown.
Bulk flow, which transports nutrients, oxygen, and water within complex multicellular organisms, also carries developmental signals. Signals carried by bulk flow can travel far greater distances through the body than signals transmitted by diffusion alone. For example, in animals the endocrine system releases hormones directly into the bloodstream, enabling them to affect cells far from those within which they formed. Thus, the sex hormones estrogen and testosterone are synthesized in reproductive organs but regulate development throughout the body, contributing to the differences between males and females. In this way, signals carried by bulk flow can induce the formation of distinct cell types and tissues along the path of signal transport.
Review Question:
What is considered to be the greatest factor that leads to cell differentiation within complex multicellular organisms?
A) the lack of oxygen in internal spaces
B) the differing environments the cells are found in
C) the three-dimensional structure of the organism
Summary
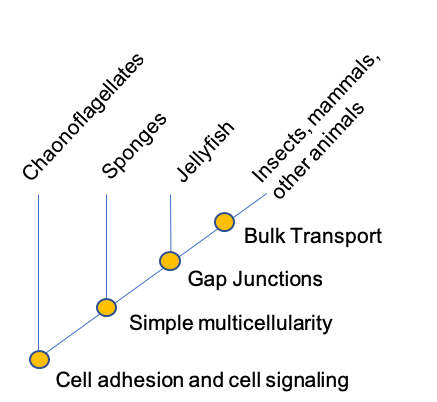
End of Section Review Questions:
2) In what order are the following thought to have evolved to have given rise to complex multicellularity?
3) How does cell differentiation differ between unicellular and complex multicellular organisms?A) Unicellular organisms do not exhibit cell differentiation
B) Multicellular organisms have a spatial differentiation of cells
C) Unicellular organisms have a spatial differentiation of cells
References
Image Attribution
Extra: Review of Cellular Connections
Animal cell connections: A tight junction is a watertight seal between two adjacent animal cells. The cells are held tightly against each other by proteins (predominantly two proteins called claudins and occludins). This tight adherence prevents materials from leaking between the cells; tight junctions are typically found in epithelial tissues that line internal organs and cavities and comprise most of the skin. Also found only in animal cells are desmosomes, which act like spot welds between adjacent epithelial cells. Short proteins called cadherins in the plasma membrane connect to intermediate filaments to create desmosomes. The cadherins join two adjacent cells together and maintain the cells in a sheet-like formation in organs and tissues that stretch, like the skin, heart, and muscles. Gap junctions in animal cells are like plasmodesmata in plant cells in that they are channels between adjacent cells that allow for the transport of ions, nutrients, and other substances that enable cells to communicate. Structurally, however, gap junctions and plasmodesmata differ. Gap junctions develop when a set of six proteins (called connexins) in the plasma membrane arrange themselves in an elongated donut-like configuration called a connexon. When the pores (“doughnut holes”) of connexons in adjacent animal cells align, a channel between the two cells forms. Gap junctions are particularly important in cardiac muscle: The electrical signal for the muscle to contract is passed efficiently through gap junctions, allowing the heart muscle cells to contract in tandem.
Learning Goals
By the end of this reading you should be able to:
- Describe the shared features of complex multicellular organisms
- Compare and contrast simple vs. complex multicellular organisms
- Explain the limitations to diffusion and the role of bulk flow in complex multicellular organisms
- Discuss the means by which cell adhesion, cell communication, and cell differentiation are accomplished in complex multicellular organisms
- Predict the order of acquisition of multicellular traits and the evolutionary consequences of complex multicellularity
Introduction
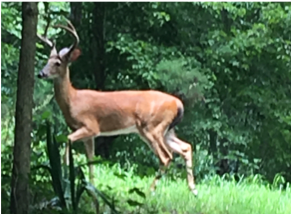
There are costs associated with multicellularity, particularly for complex multicellular organisms with differentiated reproductive tissues. In these organisms, most cells do not reproduce, instead of supporting the few that do. This requires cooperation among cells, but it creates opportunities for cells to "cheat"- to use nutrients for their own proliferation rather than the growth and reproduction of the organism as a whole. Complex multicellularity evolved at least six separate times in different eukaryotic groups; once in animal lineages, once in the green algal lineage that gave rise to land plants, twice in the fungi, once in the red algae, and once in the brown algae, producing the giant kelps that form forests in the sea.
Shared Features
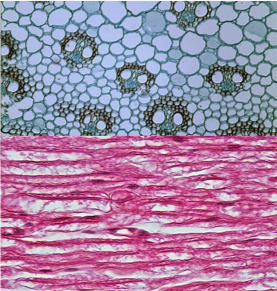
Complex multicellular organisms differ from one another in many ways, but they share three general features. (1) They have highly developed molecular mechanisms for adhesion between cells. (2) They display specialized structures that allow cells to communicate with one another. (3) They display complex patterns of cellular and tissue differentiation, guided by networks of regulatory genes. Without these features, complex multicellularity would be impossible.
For example, plants and animals both have differentiated cells and tissues with specialized functions. In plants, only some tissues photosynthesize or absorb organic molecules; other tissues transport food and oxygen through the body, and still, others generate the molecular signals that govern development. In both plants and animals, only a small subset of all cells contributes to reproduction. Because of this functional differentiation, cell or tissue loss can be lethal for the entire organism.
There is one more feature of complex multicellular organisms that is key to understanding their biology: They have a three dimensional organization, so only some cells are in direct contact with the environment. Cells that are buried within tissues, relatively far from the exterior of the organism, do not have direct access to nutrients or oxygen. Therefore, interior cells cannot grow as fast as surface cells unless there is a way to transfer resources from one part of the body to another. Similarly, interior cells do not receive signals directly from the environment, even though all cells must be able to respond to environmental signals if the organism is to grow, reproduce, and survive. Complex multicellular organisms, therefore, require mechanisms for transferring environmental signals received by cells at the body's surface to interior cells, where genes will be activated or repressed in response. Thus growth and development in complex multicellular organisms can be defined as increasing or decreasing gene expression in response to molecular signals from surrounding cells.
Review Question:
What are the characteristics that are found in both simple colonies of cells and complex multicellular organisms?
A) the ability of cells to communicate
Diffusion and Bulk Flow
A key functional challenge of complex multicellularity is transporting food, oxygen, and molecular signals rapidly across large distances within the body. How does oxygen get from the air in your lungs into your bloodstream? How does atmospheric carbon dioxide get into leaves? How does ammonia get from seawater into the cells of seaweeds? The answer to all three questions is the same: by diffusion. But oxygen absorbed by your lungs doesn't reach your toes by diffusion alone-it is transported actively, and in bulk, by blood pumped through your circulatory system. Water does not get from the roots to the leaves of plants by diffusion either, there are mechanisms at work to move it actively as well. For complex multicellular organisms to function, bulk flow of oxygen, nutrients, and signaling molecules, at rates and across distances far larger than can be achieved by diffusion alone is needed.
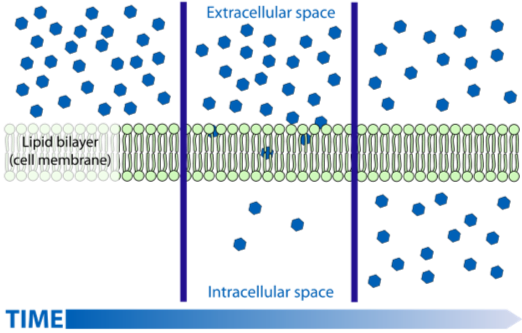
So why is diffusion effective only over short distances? Diffusion is the random motion of molecules, with net movement from areas of higher to lower concentration. The distance and speed at which molecules can diffuse depends on several factors: (1) how great the concentration difference is, large larger the differences the faster the diffusion (2) distance between concentration differences, the closer the two concentrations the faster the rate of diffusion (3) the size of the molecule, smaller molecules tend to diffuse faster than larger ones, and (4) the nature of the medium the molecule is diffusing in; molecules diffuse faster if the medium is less thick (think air vs. water). Because diffusion supplies key molecules for metabolism, it exerts a strong constraint on the size, shape, and function of cells and ultimately how eukaryotic organisms function.
Let's use oxygen as an example. Most eukaryotes require oxygen for respiration. If the cells within a tissue must rely on diffusion for their individual oxygen supply, the thickness of the tissue is limited by the concentration difference in oxygen between the cells in the tissue and the surrounding environment. The concentration difference between the two areas depends on the amount of oxygen in the environment and the rate at which oxygen is used for respiration inside the organism. The distance between the environment and the cell that is using oxygen also plays a role.
Review Question:
Which of these would increase the rate of diffusion of a molecule?
A) a high concentration gradient between two areas involved
Organisms can achieve larger sizes by circumventing limits imposed by diffusion.
Sponges can reach overall dimensions of a meter or more, but they actually consist of only a few types of cells that line a dense network of pores and canals and so remain in close contact with circulating seawater. The large size of a sponge is therefore achieved without placing metabolically active cells at any great distance from their environment. Similarly, in jellyfish, active metabolism is confined to thin tissues that line the inner and outer surfaces of the body. Essentially, a large flat surface is folded up to produce a three-dimensional structure. An increase in the surface area of a cell and or structure increases the area that is capable of interacting with the environment and ultimately the number of needed molecules to which a cell or structure has access.
Structures specialized for bulk flow
Bulk flow is any means by which molecules move through organisms at rates beyond those possible by diffusion across a concentration gradient. In humans and other vertebrate animals, the active pumping of blood through blood vessels supplies oxygen to tissues that may be more than a meter distance from the lungs. Our lungs gather the oxygen we need for respiration, but the lung is a prime example of diffusion in action, not a means of avoiding it. Because lung tissues have a very high ratio of surface area to volume, oxygen can diffuse efficiently from the air you breathe into lung tissue. A great deal of oxygen can be taken in this way, but how does it get from the lungs to our brains or toes? The distances are far too large for diffusion to be effective.
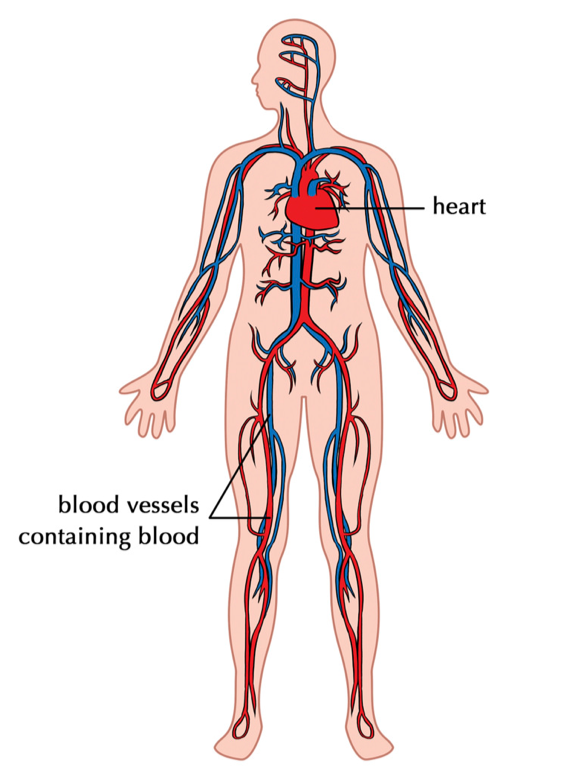
The answer is that oxygen binds to molecules of hemoglobin in red blood cells and then is carried through the bloodstream to distant sites of respiration. We circumvent diffusion by actively pumping oxygen-rich blood through our bodies. Most invertebrate animals lack well-defined blood vessels instead they have other mechanisms that circulate fluids freely throughout the body cavity. Indeed, without a mechanism like bulk flow, animals could not have achieved the range of size, shape, and function familiar to us.
Complex organisms other than animals also rely on bulk flow. A redwood tree must transport water upward from its roots to leaves that may be 100 m above the soil. If plants relied on diffusion to transport water, they would be only a few millimeters tall. How, then, do they move water? Plants move water by bulk flow through a system of specialized tissues powered by the evaporation of water from leaf surfaces. Vascular plants also have specialized tissues for the transport of nutrients and signaling molecules upward and downward through roots, stems, and leaves.
Multicellular fungi transport nutrients through networks of filaments that may be meters long, relying on osmosis to pump materials from sites of absorption to sites of metabolism. The giant kelps have an internal network of tubular cells that transports molecules through a body that can be tens of meters long. In general, when some cells within an organism are buried within tissues, far from the external environment, bulk flow is required to supply those cells with molecules needed for metabolism.
Other requirements for complex multicellular life
In addition to a means to move needed molecules over long distances and in sufficient concentration, there are three other general requirements for complex multicellular life: (1)Cells must stick together; (2) they must communicate with one another, and (3) they must participate in a network of genetic interactions that regulates cell division and differentiation. Once these are in place, the stage is set for the evolution of specialized tissues and organs in complex multicellular organisms.
Adhesion between cells
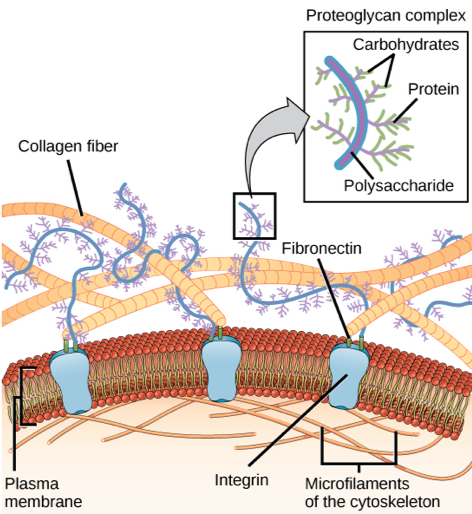
If a fertilized egg is to develop into a complex multicellular organism, it must divide many times, and the cells produced from those divisions must stick together. In addition, they must retain a specific spatial relationship with one another in order for the developing organism to function. Most animal cells release materials into the extracellular space. The primary components of these materials are proteins, and the most abundant protein is collagen. Collagen fibers are interwoven with carbohydrate-containing protein molecules called proteoglycans. Some cells attach themselves to this matrix by means of other transmembrane proteins called integrins and transmembrane proteins, especially cadherins, can also be involved in the formation of molecular attachments between cells. The extracellular matrix also allows the cells within the tissue to communicate with each other.
In plants, the cell wall connects cells together into tissues. The plant cell wall is composed predominately of cellulose fibers which are embedded in a matrix of pectin and hemicellulose. These molecules allow plant cells to attach to each other to create the complex tissue structures that support the overall plant structure. Fungi also have cell walls, although they are composed of chitin rather than cellulose, that are involved in connecting the cells together into multicellular structures.
Communication between cells
In complex multicellular organisms, it is not sufficient for cells to adhere to one another. They must also be able to communicate. Communication is important during development, guiding the patterns of gene expression that differentiate cells, tissues, and organs. The functional integration of cells within tissues and tissues within organs also depends on the flow of information among cells. Cells communicate using signaling molecules (generally a protein) synthesized by one cell which binds with a receptor protein on the surface of a second cell. This interaction can essentially flip a molecular switch that activates or represses gene expression in the receptor cell's nucleus or stimulates another response from the receiving cells. Molecular evidence indicates that many of the signaling pathways used for communication between cells in complex multicellular organisms first evolved in single-celled eukaryotes.
Thinking Question:
What function might molecular signals and receptors have had in the ancestors of complex organisms?
All cells have transmembrane receptors that respond to signals from the environment. In some cases, the signal is a molecule released by a food organism (such as the bacteria that induce simple multicellularity in choanoflagellates), and in some cases the cells sense nutrients, temperature, or oxygen level. Single celled eukaryotes also communicate with other cells within the same species, for example, to ensure that two cells can find each other to fuse in sexual reproduction. Signaling between two cells within a complex multicellular organism (plant, animal, or fungal) can be seen as a variation on this more general theme of a cell responding to other cells and the physical environment.
While all eukaryotic cells have molecular mechanisms for communication between cells, complex multicellular organisms have distinct cellular pathways for the movement of molecules from one cell to another. For example, animals more complex than sponges have gap junctions, protein channels that allow ions and signaling molecules to move from one cell into another. Gap junctions not only help cells to communicate with their neighbors, they allow targeted communication between a cell and specific cells adjacent to it.
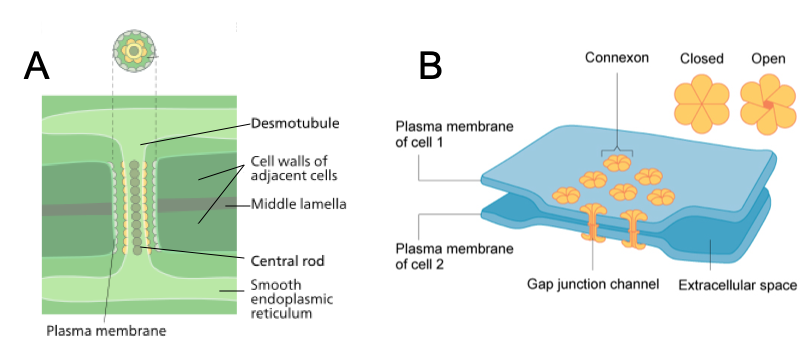
Plants, in contrast, have intercellular channels are lined by extensions of the cell membrane. Tubules running through these channels connect the endomembrane systems of the two cells. Like gap junctions, plasmodesmata permit signaling molecules to pass between cells in such a way that they can be targeted to only one or a few adjacent cells. Complex red and brown algae also have plasmodesmata, and complex fungi have pores between cells that enable communication by means of cytoplasmic flow. As similar channels do not occur in most other eukaryotic organisms, they appear to represent an important step in the evolution of complex multicellularity.
Review Question:
Genetic program for coordinated growth and cell differentiation
In multicellular organisms, growth and development is the result of molecular communication between cells. Cells have different fates depending on which genes are switched off or on, and genes are switched off and on by the molecular signals that cells receive. A signal commonly alters the production of proteins-inducing the reorganization of the cytoskeleton, for example. As a result, a stem cell may become an epithelial cell, or a muscle cell, or a neuron. This observation leads to another question: What causes the same gene to be turned on in one cell and off in another? The ultimate answer is that two cells in the same developing organism can be exposed to very different environments.
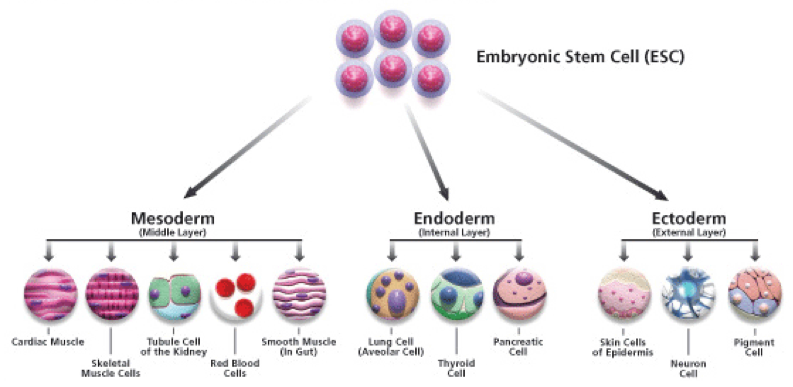
When we think about development as a process of programmed cell division and differentiation, the link to unicellular ancestors becomes clearer. Many biological innovations accompanied the evolution of complex multicellularity, but the differentiation of distinct cell types is not one of them. Many unicellular organisms have life cycles in which different cell types alternate in time, depending on environmental conditions. For example, if we experimentally starve dinoflagellate cells, two cells undergo sexual fusion to form morphologically and physiologically distinct resting cells protected by thick walls. That is, a nutrient shortage induces a change in gene expression that leads to the formation of resting cells. When food becomes available again, the cells undergo a meiotic cell division to form new feeding cells. Many other single-celled eukaryotes form resting cells in response to environmental cues, especially deprivation of nutrients or oxygen.
The innovation of complex multicellularity was to differentiate cells in space instead of time. In a three-dimensional multicellular organism, only surface cells are in direct contact with the outside environment. Interior cells are exposed to a different physical and chemical environment because nutrients, oxygen, and the light become less abundant with increasing depth within tissues. In effect, there is a gradient of environmental signals within multicellular organisms. We might, therefore, hypothesize that in the earliest organisms with three-dimensional multicellularity, a nutrient or oxygen gradient triggered oxygen- or nutrient-starved interior cells to differentiate, much as happens to trigger the formation of resting cells in their single-celled relatives. With increasing genetic control of cellular responses to signaling gradients, the seeds of complex development were sown.
Bulk flow, which transports nutrients, oxygen, and water within complex multicellular organisms, also carries developmental signals. Signals carried by bulk flow can travel far greater distances through the body than signals transmitted by diffusion alone. For example, in animals the endocrine system releases hormones directly into the bloodstream, enabling them to affect cells far from those within which they formed. Thus, the sex hormones estrogen and testosterone are synthesized in reproductive organs but regulate development throughout the body, contributing to the differences between males and females. In this way, signals carried by bulk flow can induce the formation of distinct cell types and tissues along the path of signal transport.
Review Question:
What is considered to be the greatest factor that leads to cell differentiation within complex multicellular organisms?
A) the lack of oxygen in internal spaces
B) the differing environments the cells are found in
C) the three-dimensional structure of the organism
Summary
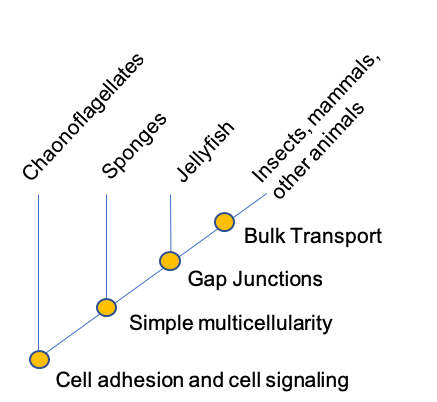
End of Section Review Questions:
2) In what order are the following thought to have evolved to have given rise to complex multicellularity?
3) How does cell differentiation differ between unicellular and complex multicellular organisms?A) Unicellular organisms do not exhibit cell differentiation
B) Multicellular organisms have a spatial differentiation of cells
C) Unicellular organisms have a spatial differentiation of cells
References
Image Attribution
Extra: Review of Cellular Connections
Animal cell connections: A tight junction is a watertight seal between two adjacent animal cells. The cells are held tightly against each other by proteins (predominantly two proteins called claudins and occludins). This tight adherence prevents materials from leaking between the cells; tight junctions are typically found in epithelial tissues that line internal organs and cavities and comprise most of the skin. Also found only in animal cells are desmosomes, which act like spot welds between adjacent epithelial cells. Short proteins called cadherins in the plasma membrane connect to intermediate filaments to create desmosomes. The cadherins join two adjacent cells together and maintain the cells in a sheet-like formation in organs and tissues that stretch, like the skin, heart, and muscles. Gap junctions in animal cells are like plasmodesmata in plant cells in that they are channels between adjacent cells that allow for the transport of ions, nutrients, and other substances that enable cells to communicate. Structurally, however, gap junctions and plasmodesmata differ. Gap junctions develop when a set of six proteins (called connexins) in the plasma membrane arrange themselves in an elongated donut-like configuration called a connexon. When the pores (“doughnut holes”) of connexons in adjacent animal cells align, a channel between the two cells forms. Gap junctions are particularly important in cardiac muscle: The electrical signal for the muscle to contract is passed efficiently through gap junctions, allowing the heart muscle cells to contract in tandem.
Learning Goals
By the end of this reading you should be able to:
- Identify the shared characteristics of fungi
- Describe the composition of the mycelium
- Describe the mode of nutrition of fungi
- Explain sexual and asexual reproduction in fungi
Introduction
The word fungus comes from the Latin word for mushrooms. Indeed, the familiar mushroom is a reproductive structure used by many types of fungi. However, there are also many fungi species that don't produce mushrooms at all. While a typical fungal cell contains a true nucleus and many membrane-bound organelles, within the kingdom Fungi there is an enormous variety of living organisms collectively referred to as Eucomycota, or true Fungi. Scientists have identified about 100,000 species of fungi but this is only a fraction of the 1.5 million species of fungus likely present on Earth. Edible mushrooms, yeasts, black mold, and the producer of the antibiotic penicillin, Penicillium notatum, are all members of the kingdom Fungi, which belongs to the domain Eukarya.
Fungal Characteristics
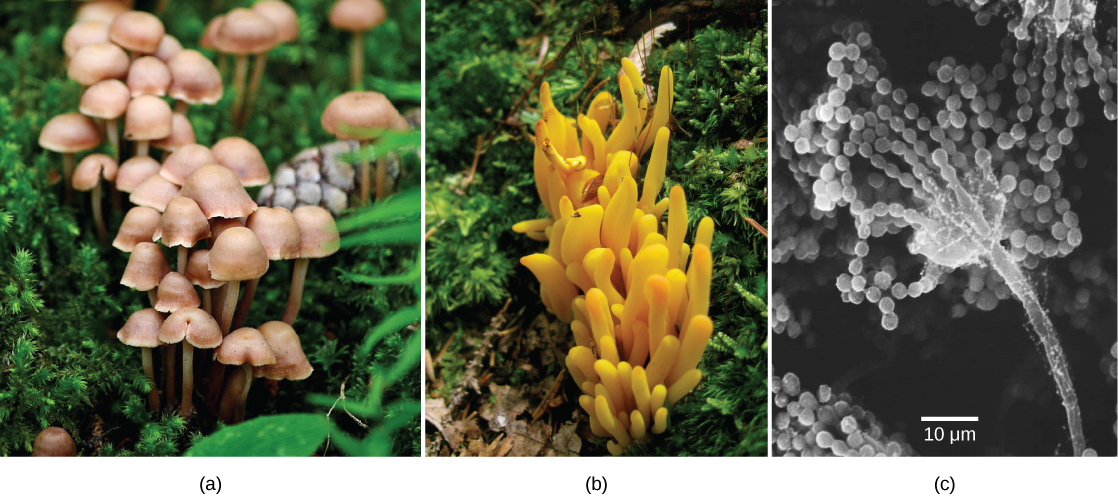
Fungi, once considered plant-like organisms, are actually more closely related to animals than plants. Like animals, fungi are not capable of photosynthesis and therefore are heterotrophic.
Some fungal organisms multiply only asexually, whereas others undergo both asexual reproduction and sexual reproduction with a form of alternation of generations. Most fungi produce a large number of spores, which are haploid cells that can undergo mitosis to form multicellular, haploid individuals. Like bacteria, fungi play an essential role in ecosystems because they are decomposers and participate in the cycling of nutrients by breaking down organic materials into simple molecules (Fig. 1).
Fungi often interact with other organisms, forming beneficial associations. For example, most terrestrial plants form mutualistic relationships with fungi. The roots of the plant connect with the underground parts of the fungus forming mycorrhizae. Through mycorrhizae, the fungus and plant exchange nutrients and water, greatly aiding the survival of both species. Alternatively, lichens are an association between a fungus and its photosynthetic partner (usually an alga). Fungi also cause serious infections in plants and animals. For example, Dutch elm disease, which is caused by the fungus Ophiostoma ulmi, is a particularly devastating type of fungal infestation that destroys many native species of elm (Ulmus sp.) by infecting the tree’s vascular system. The elm bark beetle acts as a vector, transmitting the disease from tree to tree. Accidentally introduced in the 1900s, the fungus decimated elm trees across the continent. Many European and Asiatic elms are less susceptible to Dutch elm disease than American elms.
Thinking Question:
In humans, fungal infections are generally considered challenging to treat. Unlike bacteria, fungi do not respond to traditional antibiotic therapy, since they are eukaryotes. Fungal infections may prove deadly for individuals with compromised immune systems.
Fungi have many commercial applications. The food industry uses yeasts in baking, brewing, and cheese and wine production. Many industrial compounds are byproducts of fungal fermentation. Fungi are the source of many commercial enzymes and antibiotics.
Although humans have used yeasts and mushrooms since prehistoric times until recently the biology of fungi was poorly understood. Up until the mid-20th century, many scientists classified fungi as plants. Fungi, like plants, arose mostly sessile and seemingly rooted in place. They possess a stem-like structure similar to plants, as well as having a root-like fungal mycelium in the soil. In addition, their mode of nutrition was poorly understood. Progress in the field of fungal biology was the result of mycology: the scientific study of fungi. Based on fossil evidence, fungi appeared in the pre-Cambrian era, about 450 million years ago. Molecular biology analysis of the fungal genome demonstrates that fungi are more closely related to animals than plants. They are a polyphyletic group of organisms that share characteristics, rather than sharing a single common ancestor.
Cell Structure and Function
Fungi are eukaryotes, and as such, have a complex cellular organization. As eukaryotes, fungal cells contain a membrane-bound nucleus. The DNA in the nucleus is wrapped around histone proteins, as is observed in other eukaryotic cells. A few types of fungi have structures comparable to bacterial plasmids (loops of DNA); however, the horizontal transfer of genetic information from one mature bacterium to another rarely occurs in fungi. Fungal cells also contain mitochondria and a complex system of internal membranes, including the endoplasmic reticulum and Golgi apparatus.

Unlike plant cells, fungal cells do not have chloroplasts or chlorophyll. Many fungi display bright colors arising from other cellular pigments, ranging from red to green to black. The poisonous Amanita muscaria (fly agaric) is recognizable by its bright red cap with white patches (Fig. 2). Pigments in fungi are associated with the cell wall and play a protective role against ultraviolet radiation. Some fungal pigments are toxic.
Like plant cells, fungal cells have a thick cell wall. The rigid layers of fungal cell walls contain complex polysaccharides called chitin and glucans. Chitin, also found in the exoskeleton of insects, gives structural strength to the cell walls of fungi. The wall protects the cell from desiccation and predators. Fungi have plasma membranes similar to other eukaryotes, except that the structure is stabilized by ergosterol: a steroid molecule that replaces the cholesterol found in animal cell membranes. Most members of the kingdom Fungi are nonmotile, however, flagella are produced only by the gametes in the Phylum Chytridiomycota.
Review Question:
Growth
The vegetative body of a fungus is a unicellular or multicellular thallus. Dimorphic fungi can change from the unicellular to multicellular state depending on environmental conditions. Unicellular fungi are generally referred to as yeasts. Saccharomyces cerevisiae (baker’s yeast) and Candida species (the agents of thrush, a common fungal infection) are examples of unicellular fungi (Fig. 3).

Most fungi are multicellular organisms. They display two distinct morphological stages: vegetative and reproductive. The vegetative stage consists of a tangle of slender thread-like structures called hyphae (singular, hypha), whereas the reproductive stage can be more conspicuous. The mass of hyphae is a mycelium (Fig. 4). It can grow on a surface, in soil or decaying material, in a liquid, or even on living tissue. Although individual hyphae must be observed under a microscope, the mycelium of a fungus can be very large, with some species truly being “the fungus humongous.” The giant Armillaria solidipes (honey mushroom) is considered the largest organism on Earth, spreading across more than 2,000 acres of underground soil in eastern Oregon; it is estimated to be at least 2,400 years old.

Most fungal hyphae are divided into separate cells by endwalls called septa (singular, septum) (Fig. 5a, c). In most phyla of fungi, tiny holes in the septa allow for the rapid flow of nutrients and small molecules from cell to cell along the hypha. They are described as perforated septa. The hyphae in bread molds (which belong to the Phylum Zygomycota) are not separated by septa. Instead, they are formed by large cells containing many nuclei, an arrangement described as coenocytic (SEE-no-SI-tic) hyphae (Fig. 5b).

Fungi thrive in environments that are moist and slightly acidic and can grow with or without light. They vary in their oxygen requirement. Most fungi are obligate aerobes, requiring oxygen to survive. Other species, such as the Chytridiomycota that reside in the rumen of cattle, are obligate anaerobes, in that they only use anaerobic respiration because oxygen will disrupt their metabolism or kill them. Yeasts are intermediate, being facultative anaerobes. This means that they grow best in the presence of oxygen using aerobic respiration but can survive using anaerobic respiration when oxygen is not available. The alcohol produced from yeast fermentation is used in wine and beer production.
Review Question:
Nutrition
Fungi are heterotrophs. In addition, fungi do not fix nitrogen from the atmosphere and so must obtain it from their diet, like animals. However, unlike most animals, digestion precedes ingestion. First, exoenzymes are transported out of the hyphae, where they process nutrients in the environment. Then, the smaller molecules produced by this external digestion are absorbed through the large surface area of the mycelium. As with animal cells, the polysaccharide of storage is glycogen, rather than starch, as found in plants.
Fungi are mostly saprobes (saprophyte is an equivalent term): organisms that derive nutrients from decaying organic matter. They obtain their nutrients from dead or decomposing organic matter, mainly plant material. Fungal exoenzymes are able to break down insoluble polysaccharides, such as the cellulose and lignin of dead wood, into readily absorbable glucose molecules. The carbon, nitrogen, and other elements are thus released into the environment. Because of their varied metabolic pathways, fungi fulfill an important ecological role and are being investigated as potential tools in bioremediation. For example, some species of fungi can be used to break down diesel oil and polycyclic aromatic hydrocarbons (PAHs). Other species take up heavy metals, such as cadmium and lead.
Some fungi are parasitic, infecting either plants or animals. Smut and Dutch elm disease affect plants, whereas athlete’s foot and candidiasis (thrush) are medically important fungal infections in humans. In environments poor in nitrogen, some fungi resort to predation of nematodes (small non-segmented roundworms). Species of Arthrobotrys fungi have a number of mechanisms to trap nematodes. One mechanism involves constricting rings within the network of hyphae (Fig. 6). The rings swell when they touch the nematode, gripping it in a tight hold. The fungus penetrates the tissue of the worm by extending specialized hyphae called haustoria. Many parasitic fungi possess haustoria, as these structures penetrate the tissues of the host, release digestive enzymes within the host's body, and absorb the digested nutrients.
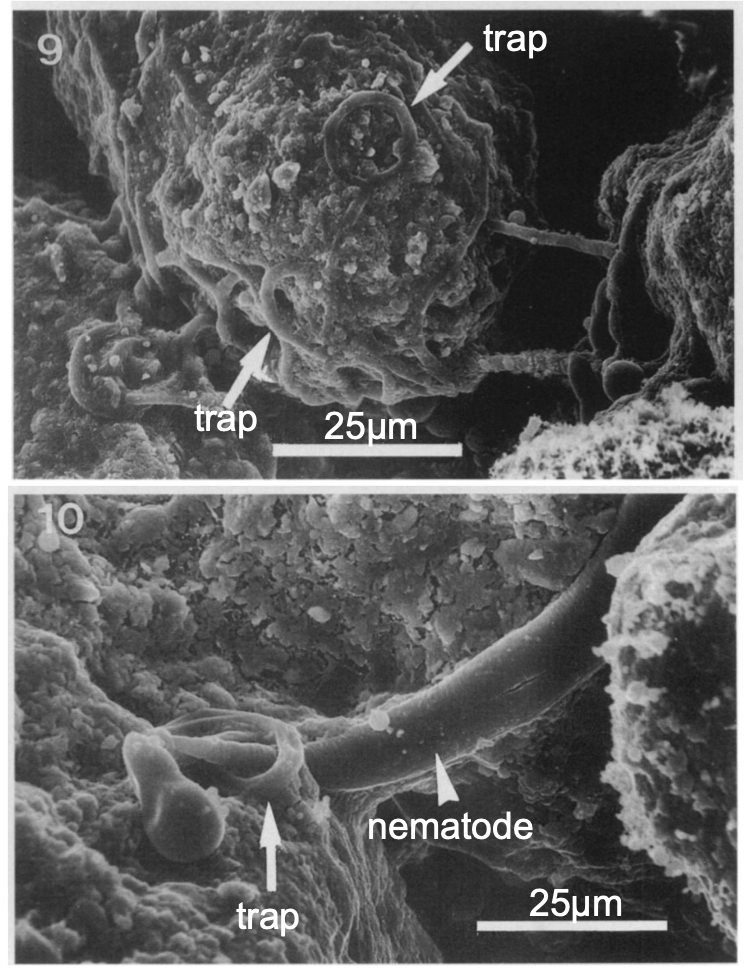
Review Question:
Reproduction
Fungi reproduce sexually and/or asexually. Perfect fungi reproduce both sexually and asexually, while the so-called imperfect fungi reproduce only asexually (by mitosis). In both sexual and asexual reproduction, fungi produce spores that disperse from the parent organism by either floating on the wind or hitching a ride on an animal. Fungal spores are smaller and lighter than plant seeds. The giant puffball mushroom bursts open and releases trillions of spores. The huge number of spores released increases the likelihood of landing in an environment that will support growth (Fig. 7).

Asexual Reproduction
Fungi reproduce asexually by fragmentation, budding, or producing spores. Fragments of hyphae can grow new colonies. Somatic cells in yeast form buds. During budding (a type of cytokinesis), a bulge forms on the side of the cell, the nucleus divides mitotically, and the bud ultimately detaches itself from the mother cell (Fig. 8).

The most common mode of asexual reproduction is through the formation of asexual spores, which are produced by one parent only (through mitosis) and are genetically identical to that parent (Fig. 9). Spores allow fungi to expand their distribution and colonize new environments. They may be released from the parent thallus either outside or within a special reproductive sac called a sporangium.

There are many types of asexual spores. Conidiospores are unicellular or multicellular spores that are released directly from the tip or side of the hypha. Other asexual spores originate in the fragmentation of a hypha to form single cells that are released as spores; some of these have a thick wall surrounding the fragment. Yet others bud off the vegetative parent cell. Sporangiospores are produced in a sporangium (Fig. 10).

Sexual Reproduction
Sexual reproduction introduces genetic variation into a population of fungi. In fungi, sexual reproduction often occurs in response to adverse environmental conditions. During sexual reproduction, two mating types are produced. When both mating types are present in the same mycelium, it is called homothallic, or self-fertile. Heterothallic mycelia require two different, but compatible, mycelia to reproduce sexually.
Although there are many variations in fungal sexual reproduction, all include the following three stages (Figure 10). First, during plasmogamy (literally, “marriage or union of cytoplasm”), two haploid cells fuse, leading to a dikaryotic stage where two haploid nuclei coexist in a single cell. During karyogamy (“nuclear marriage”), the haploid nuclei fuse to form a diploid zygote nucleus. Finally, meiosis takes place in the gametangia (singular, gametangium) organs, in which gametes of different mating types are generated. At this stage, spores are disseminated into the environment.
Review the characteristics of fungi by visiting this interactive site (http://openstaxcollege.org/l/fungi_kingdom) from Wisconsin-online.
Review Question:
SUMMARY
Fungi are eukaryotic organisms that appeared on land more than 450 million years ago. They are heterotrophs and contain neither photosynthetic pigments such as chlorophyll nor organelles such as chloroplasts. Because fungi feed on decaying and dead matter, they are saprobes. Fungi are important decomposers that release essential elements into the environment. External enzymes digest nutrients that are absorbed by the body of the fungus, which is called a thallus. A thick cell wall made of chitin surrounds the cell. Fungi can be unicellular as yeasts or develop a network of filaments called a mycelium, which is often described as mold. Most species multiply by asexual and sexual reproductive cycles and display an alternation of generations. Another group of fungi does not have a sexual cycle. Sexual reproduction involves plasmogamy (the fusion of the cytoplasm), followed by karyogamy (the fusion of nuclei). Meiosis regenerates haploid individuals, resulting in haploid spores.
End of Section Review Questions:
Review: Reproduction
Review: Nutrition
Learning Goals
By the end of this reading you should be able to:
- Identify the shared characteristics of fungi
- Describe the composition of the mycelium
- Describe the mode of nutrition of fungi
- Explain sexual and asexual reproduction in fungi
Introduction
The word fungus comes from the Latin word for mushrooms. Indeed, the familiar mushroom is a reproductive structure used by many types of fungi. However, there are also many fungi species that don't produce mushrooms at all. While a typical fungal cell contains a true nucleus and many membrane-bound organelles, within the kingdom Fungi there is an enormous variety of living organisms collectively referred to as Eucomycota, or true Fungi. Scientists have identified about 100,000 species of fungi but this is only a fraction of the 1.5 million species of fungus likely present on Earth. Edible mushrooms, yeasts, black mold, and the producer of the antibiotic penicillin, Penicillium notatum, are all members of the kingdom Fungi, which belongs to the domain Eukarya.
Fungal Characteristics
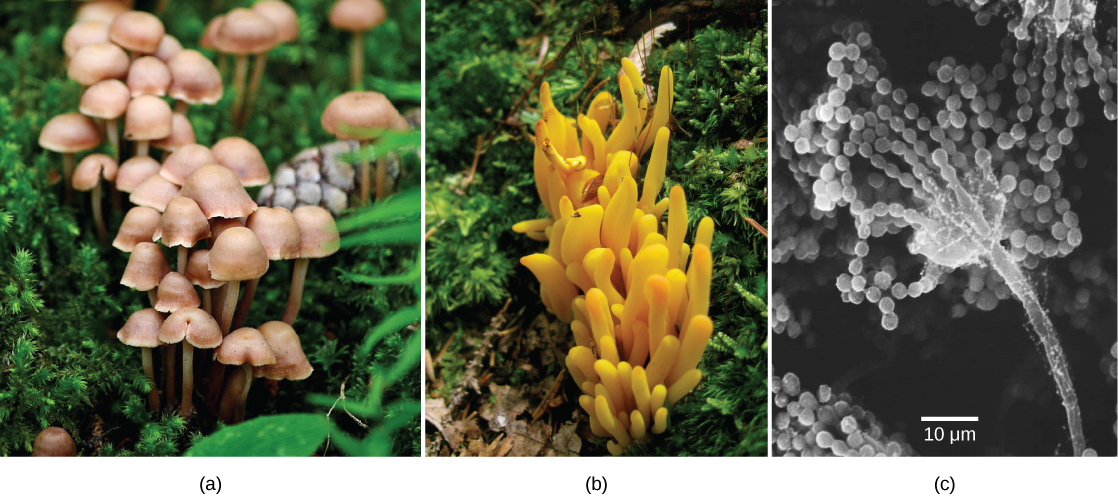
Fungi, once considered plant-like organisms, are actually more closely related to animals than plants. Like animals, fungi are not capable of photosynthesis and therefore are heterotrophic.
Some fungal organisms multiply only asexually, whereas others undergo both asexual reproduction and sexual reproduction with a form of alternation of generations. Most fungi produce a large number of spores, which are haploid cells that can undergo mitosis to form multicellular, haploid individuals. Like bacteria, fungi play an essential role in ecosystems because they are decomposers and participate in the cycling of nutrients by breaking down organic materials into simple molecules (Fig. 1).
Fungi often interact with other organisms, forming beneficial associations. For example, most terrestrial plants form mutualistic relationships with fungi. The roots of the plant connect with the underground parts of the fungus forming mycorrhizae. Through mycorrhizae, the fungus and plant exchange nutrients and water, greatly aiding the survival of both species. Alternatively, lichens are an association between a fungus and its photosynthetic partner (usually an alga). Fungi also cause serious infections in plants and animals. For example, Dutch elm disease, which is caused by the fungus Ophiostoma ulmi, is a particularly devastating type of fungal infestation that destroys many native species of elm (Ulmus sp.) by infecting the tree’s vascular system. The elm bark beetle acts as a vector, transmitting the disease from tree to tree. Accidentally introduced in the 1900s, the fungus decimated elm trees across the continent. Many European and Asiatic elms are less susceptible to Dutch elm disease than American elms.
Thinking Question:
In humans, fungal infections are generally considered challenging to treat. Unlike bacteria, fungi do not respond to traditional antibiotic therapy, since they are eukaryotes. Fungal infections may prove deadly for individuals with compromised immune systems.
Fungi have many commercial applications. The food industry uses yeasts in baking, brewing, and cheese and wine production. Many industrial compounds are byproducts of fungal fermentation. Fungi are the source of many commercial enzymes and antibiotics.
Although humans have used yeasts and mushrooms since prehistoric times until recently the biology of fungi was poorly understood. Up until the mid-20th century, many scientists classified fungi as plants. Fungi, like plants, arose mostly sessile and seemingly rooted in place. They possess a stem-like structure similar to plants, as well as having a root-like fungal mycelium in the soil. In addition, their mode of nutrition was poorly understood. Progress in the field of fungal biology was the result of mycology: the scientific study of fungi. Based on fossil evidence, fungi appeared in the pre-Cambrian era, about 450 million years ago. Molecular biology analysis of the fungal genome demonstrates that fungi are more closely related to animals than plants. They are a polyphyletic group of organisms that share characteristics, rather than sharing a single common ancestor.
Cell Structure and Function
Fungi are eukaryotes, and as such, have a complex cellular organization. As eukaryotes, fungal cells contain a membrane-bound nucleus. The DNA in the nucleus is wrapped around histone proteins, as is observed in other eukaryotic cells. A few types of fungi have structures comparable to bacterial plasmids (loops of DNA); however, the horizontal transfer of genetic information from one mature bacterium to another rarely occurs in fungi. Fungal cells also contain mitochondria and a complex system of internal membranes, including the endoplasmic reticulum and Golgi apparatus.

Unlike plant cells, fungal cells do not have chloroplasts or chlorophyll. Many fungi display bright colors arising from other cellular pigments, ranging from red to green to black. The poisonous Amanita muscaria (fly agaric) is recognizable by its bright red cap with white patches (Fig. 2). Pigments in fungi are associated with the cell wall and play a protective role against ultraviolet radiation. Some fungal pigments are toxic.
Like plant cells, fungal cells have a thick cell wall. The rigid layers of fungal cell walls contain complex polysaccharides called chitin and glucans. Chitin, also found in the exoskeleton of insects, gives structural strength to the cell walls of fungi. The wall protects the cell from desiccation and predators. Fungi have plasma membranes similar to other eukaryotes, except that the structure is stabilized by ergosterol: a steroid molecule that replaces the cholesterol found in animal cell membranes. Most members of the kingdom Fungi are nonmotile, however, flagella are produced only by the gametes in the Phylum Chytridiomycota.
Review Question:
Growth
The vegetative body of a fungus is a unicellular or multicellular thallus. Dimorphic fungi can change from the unicellular to multicellular state depending on environmental conditions. Unicellular fungi are generally referred to as yeasts. Saccharomyces cerevisiae (baker’s yeast) and Candida species (the agents of thrush, a common fungal infection) are examples of unicellular fungi (Fig. 3).

Most fungi are multicellular organisms. They display two distinct morphological stages: vegetative and reproductive. The vegetative stage consists of a tangle of slender thread-like structures called hyphae (singular, hypha), whereas the reproductive stage can be more conspicuous. The mass of hyphae is a mycelium (Fig. 4). It can grow on a surface, in soil or decaying material, in a liquid, or even on living tissue. Although individual hyphae must be observed under a microscope, the mycelium of a fungus can be very large, with some species truly being “the fungus humongous.” The giant Armillaria solidipes (honey mushroom) is considered the largest organism on Earth, spreading across more than 2,000 acres of underground soil in eastern Oregon; it is estimated to be at least 2,400 years old.

Most fungal hyphae are divided into separate cells by endwalls called septa (singular, septum) (Fig. 5a, c). In most phyla of fungi, tiny holes in the septa allow for the rapid flow of nutrients and small molecules from cell to cell along the hypha. They are described as perforated septa. The hyphae in bread molds (which belong to the Phylum Zygomycota) are not separated by septa. Instead, they are formed by large cells containing many nuclei, an arrangement described as coenocytic (SEE-no-SI-tic) hyphae (Fig. 5b).

Fungi thrive in environments that are moist and slightly acidic and can grow with or without light. They vary in their oxygen requirement. Most fungi are obligate aerobes, requiring oxygen to survive. Other species, such as the Chytridiomycota that reside in the rumen of cattle, are obligate anaerobes, in that they only use anaerobic respiration because oxygen will disrupt their metabolism or kill them. Yeasts are intermediate, being facultative anaerobes. This means that they grow best in the presence of oxygen using aerobic respiration but can survive using anaerobic respiration when oxygen is not available. The alcohol produced from yeast fermentation is used in wine and beer production.
Review Question:
Nutrition
Fungi are heterotrophs. In addition, fungi do not fix nitrogen from the atmosphere and so must obtain it from their diet, like animals. However, unlike most animals, digestion precedes ingestion. First, exoenzymes are transported out of the hyphae, where they process nutrients in the environment. Then, the smaller molecules produced by this external digestion are absorbed through the large surface area of the mycelium. As with animal cells, the polysaccharide of storage is glycogen, rather than starch, as found in plants.
Fungi are mostly saprobes (saprophyte is an equivalent term): organisms that derive nutrients from decaying organic matter. They obtain their nutrients from dead or decomposing organic matter, mainly plant material. Fungal exoenzymes are able to break down insoluble polysaccharides, such as the cellulose and lignin of dead wood, into readily absorbable glucose molecules. The carbon, nitrogen, and other elements are thus released into the environment. Because of their varied metabolic pathways, fungi fulfill an important ecological role and are being investigated as potential tools in bioremediation. For example, some species of fungi can be used to break down diesel oil and polycyclic aromatic hydrocarbons (PAHs). Other species take up heavy metals, such as cadmium and lead.
Some fungi are parasitic, infecting either plants or animals. Smut and Dutch elm disease affect plants, whereas athlete’s foot and candidiasis (thrush) are medically important fungal infections in humans. In environments poor in nitrogen, some fungi resort to predation of nematodes (small non-segmented roundworms). Species of Arthrobotrys fungi have a number of mechanisms to trap nematodes. One mechanism involves constricting rings within the network of hyphae (Fig. 6). The rings swell when they touch the nematode, gripping it in a tight hold. The fungus penetrates the tissue of the worm by extending specialized hyphae called haustoria. Many parasitic fungi possess haustoria, as these structures penetrate the tissues of the host, release digestive enzymes within the host's body, and absorb the digested nutrients.
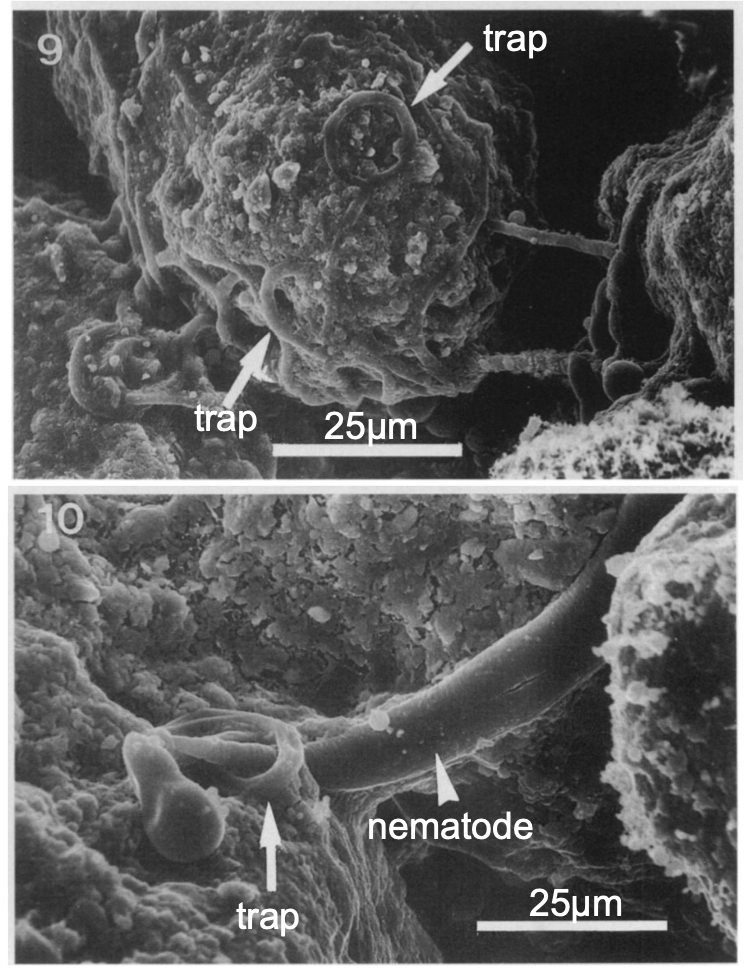
Review Question:
Reproduction
Fungi reproduce sexually and/or asexually. Perfect fungi reproduce both sexually and asexually, while the so-called imperfect fungi reproduce only asexually (by mitosis). In both sexual and asexual reproduction, fungi produce spores that disperse from the parent organism by either floating on the wind or hitching a ride on an animal. Fungal spores are smaller and lighter than plant seeds. The giant puffball mushroom bursts open and releases trillions of spores. The huge number of spores released increases the likelihood of landing in an environment that will support growth (Fig. 7).

Asexual Reproduction
Fungi reproduce asexually by fragmentation, budding, or producing spores. Fragments of hyphae can grow new colonies. Somatic cells in yeast form buds. During budding (a type of cytokinesis), a bulge forms on the side of the cell, the nucleus divides mitotically, and the bud ultimately detaches itself from the mother cell (Fig. 8).

The most common mode of asexual reproduction is through the formation of asexual spores, which are produced by one parent only (through mitosis) and are genetically identical to that parent (Fig. 9). Spores allow fungi to expand their distribution and colonize new environments. They may be released from the parent thallus either outside or within a special reproductive sac called a sporangium.

There are many types of asexual spores. Conidiospores are unicellular or multicellular spores that are released directly from the tip or side of the hypha. Other asexual spores originate in the fragmentation of a hypha to form single cells that are released as spores; some of these have a thick wall surrounding the fragment. Yet others bud off the vegetative parent cell. Sporangiospores are produced in a sporangium (Fig. 10).

Sexual Reproduction
Sexual reproduction introduces genetic variation into a population of fungi. In fungi, sexual reproduction often occurs in response to adverse environmental conditions. During sexual reproduction, two mating types are produced. When both mating types are present in the same mycelium, it is called homothallic, or self-fertile. Heterothallic mycelia require two different, but compatible, mycelia to reproduce sexually.
Although there are many variations in fungal sexual reproduction, all include the following three stages (Figure 10). First, during plasmogamy (literally, “marriage or union of cytoplasm”), two haploid cells fuse, leading to a dikaryotic stage where two haploid nuclei coexist in a single cell. During karyogamy (“nuclear marriage”), the haploid nuclei fuse to form a diploid zygote nucleus. Finally, meiosis takes place in the gametangia (singular, gametangium) organs, in which gametes of different mating types are generated. At this stage, spores are disseminated into the environment.
Review the characteristics of fungi by visiting this interactive site (http://openstaxcollege.org/l/fungi_kingdom) from Wisconsin-online.
Review Question:
SUMMARY
Fungi are eukaryotic organisms that appeared on land more than 450 million years ago. They are heterotrophs and contain neither photosynthetic pigments such as chlorophyll nor organelles such as chloroplasts. Because fungi feed on decaying and dead matter, they are saprobes. Fungi are important decomposers that release essential elements into the environment. External enzymes digest nutrients that are absorbed by the body of the fungus, which is called a thallus. A thick cell wall made of chitin surrounds the cell. Fungi can be unicellular as yeasts or develop a network of filaments called a mycelium, which is often described as mold. Most species multiply by asexual and sexual reproductive cycles and display an alternation of generations. Another group of fungi does not have a sexual cycle. Sexual reproduction involves plasmogamy (the fusion of the cytoplasm), followed by karyogamy (the fusion of nuclei). Meiosis regenerates haploid individuals, resulting in haploid spores.
End of Section Review Questions:
Review: Reproduction
Review: Nutrition