4
Learning Goals
By the end of this reading you should be able to:
-
Describe the different types of variation in a population
-
Explain why only heritable variation can be acted upon by natural selection
-
Describe genetic drift and the bottleneck effect
-
Explain how each evolutionary force can influence the allele frequencies of a population
Introduction
Genetic Variance
Heritability is the fraction of phenotype variation that can be attributed to genetic differences, or genetic variance, among individuals in a population. The greater the hereditability of a population’s phenotypic variation, the more susceptible it is to the evolutionary forces that act on heritable variation.
The diversity of alleles and genotypes within a population is called genetic variance. When scientists are involved in the breeding of a species, such as with animals in zoos and nature preserves, they try to increase a population’s genetic variance to preserve as much of the phenotypic diversity as they can. This also helps reduce the risks associated with inbreeding, the mating of closely related individuals, which can have the undesirable effect of bringing together deleterious recessive mutations that can cause abnormalities and susceptibility to disease. For example, a disease that is caused by a rare, recessive allele might exist in a population, but it will only manifest itself when an individual carries two copies of the allele. Because the allele is rare in a normal, healthy population with unrestricted habitat, the chance that two carriers will mate is low, and even then, only 25 percent of their offspring will inherit the disease allele from both parents. While it is likely to happen at some point, it will not happen frequently enough for natural selection to be able to swiftly eliminate the allele from the population, and as a result, the allele will be maintained at low levels in the gene pool. However, if a family of carriers begins to interbreed with each other, this will dramatically increase the likelihood of two carriers mating and eventually producing diseased offspring, a phenomenon known as inbreeding depression.
Evolutionary Forces
Genetic Drift
Small populations are more susceptible to the forces of genetic drift. Large populations, on the other hand, are buffered against the effects of chance. If one individual of a population of 10 individuals happens to die at a young age before it leaves any offspring to the next generation, all its genes—1/10 of the population’s gene pool—will be suddenly lost. In a population of 100, that’s only 1 percent of the overall gene pool; therefore, it is much less impactful on the population’s genetic structure (Fig. 1).
Use this link to watch an animation of random sampling and genetic drift in action.
Another scenario in which populations might experience a strong influence of genetic drift is if some portion of the population leaves to start a new population in a new location or if a population gets divided by a physical barrier of some kind. In this situation, those individuals are unlikely to be representative of the entire population, which results in the founder effect. The founder effect occurs when the genetic structure changes to match that of the new population’s founding fathers and mothers. This effect is believed to have been a key factor in the genetic history of the Afrikaner population of Dutch settlers in South Africa, as evidenced by mutations that are common in Afrikaners but rare in most other populations. This is likely since a higher-than-normal proportion of the founding colonists carried these mutations. As a result, the population expresses unusually high incidences of Huntington’s disease (HD) and Fanconi anemia (FA), a genetic disorder known to cause blood marrow and congenital abnormalities—even cancer. [2]
Thinking Question:
Do you think genetic drift would happen more quickly on an island or on the mainland?
Gene Flow
Mutation
Nonrandom Mating (Sexual Selection)
Review Question:
Environmental Variance
Summary
End of Section Review Questions:
Review: Mechanisms of Evolution
2) When male lions reach sexual maturity, they leave their group in search of a new pride. This can alter the allele frequencies of the population through which of the following mechanisms?
Review: Genetic Variation
3) Which of the following evolutionary forces can introduce new genetic variation into a population?
Review: Mating Impacts
4) When closely related individuals mate with each other or inbreed, the offspring are often not as fit as the offspring of two unrelated individuals. Why?
Attribution
Modification of OpenStax Biology 2nd Edition, Biology 2e. OpenStax CNX. Nov 26, 2018 http://cnx.org/contents/8d50a0af- 948b-4204-a71d-4826cba765b8@15.1.
Figure 1. Genetic Drift courtesy of OpenStax, Rice University / CC BY (https://creativecommons.org/licenses/by/4.0)
Figure 2. Gene Flow courtesy of Tsaneda / CC BY (https://creativecommons.org/licenses/by/3.0)
Figure 3. Temperature-dependent sex determination. Left image courtesy of Planetseeker / CC BY-SA (https://creativecommons.org/licenses/by-sa/4.0). Right image courtesy of: Steve Hillebrand, USFWS Public Domain.
Learning Goals:
By the end of this reading you should be able to:
- Describe the different means by which animals exchange gases
- Explain the role that diffusion plays in different systems of gas exchange
- Describe the process of countercurrent gas exchange
- Compare and contrast the tracheal system of insects with the mammalian respiratory system
Introduction
The primary function of the respiratory system is to deliver oxygen to the cells of the body’s tissues and remove carbon dioxide, a cell waste product. All aerobic organisms require oxygen to carry out their metabolic functions. Along the evolutionary tree, different organisms have devised different means of obtaining oxygen from the surrounding atmosphere. As with other organisms, the environment in which the animal lives greatly determines how an animal respires. In addition, the complexity of the respiratory system usually correlates with the size of the organism. As animal size increases, diffusion distances increase and the ratio of surface area to volume drops. In unicellular organisms, diffusion across the cell membrane is sufficient for supplying oxygen to the cell. However, diffusion is a slow, passive transport process. In order for diffusion to be a feasible means of providing oxygen to the cell, the rate of oxygen uptake must match the rate of diffusion across the membrane. In other words, if the cell were very large or thick, diffusion would not be able to provide oxygen quickly enough to the inside of the cell. Therefore, dependence on diffusion as a means of obtaining oxygen and removing carbon dioxide remains feasible only for small organisms or those with highly-flattened bodies, such as many flatworms (Platyhelminthes). Larger organisms had to evolve specialized respiratory tissues, such as gills, lungs, and respiratory passages accompanied by complex circulatory systems, to transport oxygen throughout their entire body.
Direct Diffusion
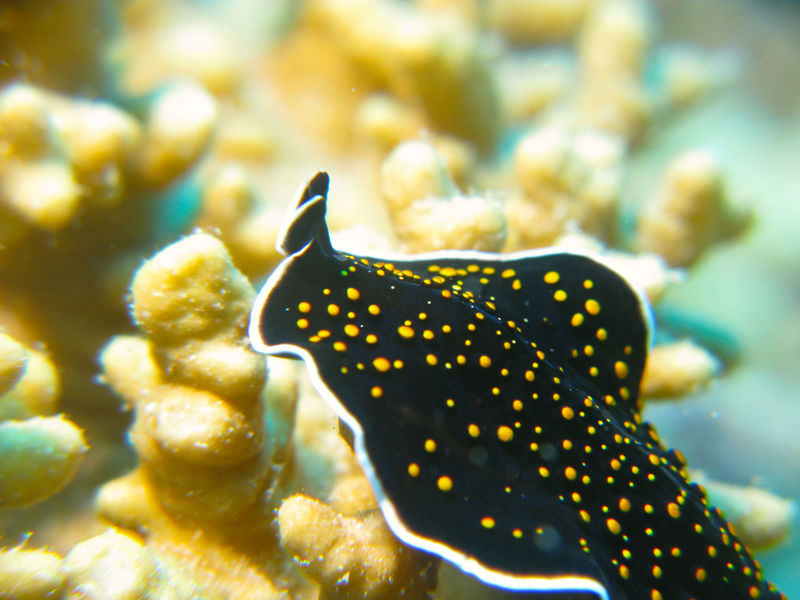
For many small multicellular organisms (less than 1mm in diameter), diffusion across the outer membrane is sufficient to meet their oxygen needs. In simple organisms, such as cnidarians and flatworms (Fig 1), every cell in the body is close to the external environment. Thus the cells are kept moist and gases can diffuse quickly via direct diffusion. Flatworms are small, literally flatworms, which ‘breathe’ through diffusion across the outer membrane. The flat shape of these organisms increases the surface area for diffusion, ensuring that each cell within the body is close to the outer membrane surface and has access to oxygen. If the flatworm had a cylindrical body, then the cells in the center would not be able to get oxygen.
Skin and Gills
Earthworms and amphibians use their skin (integument) as a respiratory organ. A dense network of capillaries lies just below the skin and facilitates gas exchange between the external environment and the circulatory system. The respiratory surface must be kept moist in order for the gases to dissolve and diffuse across cell membranes.
Organisms that live in water need to obtain oxygen from the water. Oxygen dissolves in water but at a lower concentration than in the atmosphere. The atmosphere has roughly 21 percent oxygen. In water, the oxygen concentration is much smaller than that. Fish and many other aquatic organisms have evolved gills to take up the dissolved oxygen from water.
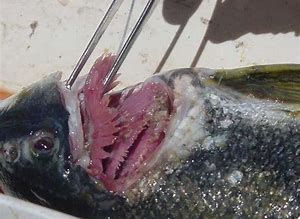
Gills are thin tissue filaments that are highly branched and folded (Fig. 2). When water passes over the gills, the dissolved oxygen in water rapidly diffuses across the gills into the bloodstream. The circulatory system can then carry the oxygenated blood to the other parts of the body. In animals that contain coelomic fluid instead of blood, oxygen diffuses across the gill surfaces into the coelomic fluid. Gills are found in mollusks, annelids, and crustaceans.
The folded surfaces of the gills provide a large surface area to ensure that the fish gets sufficient oxygen. Diffusion is a process in which material travels from regions of high concentration to low concentration until equilibrium is reached. In this case, blood with a low concentration of oxygen molecules circulates through the gills.
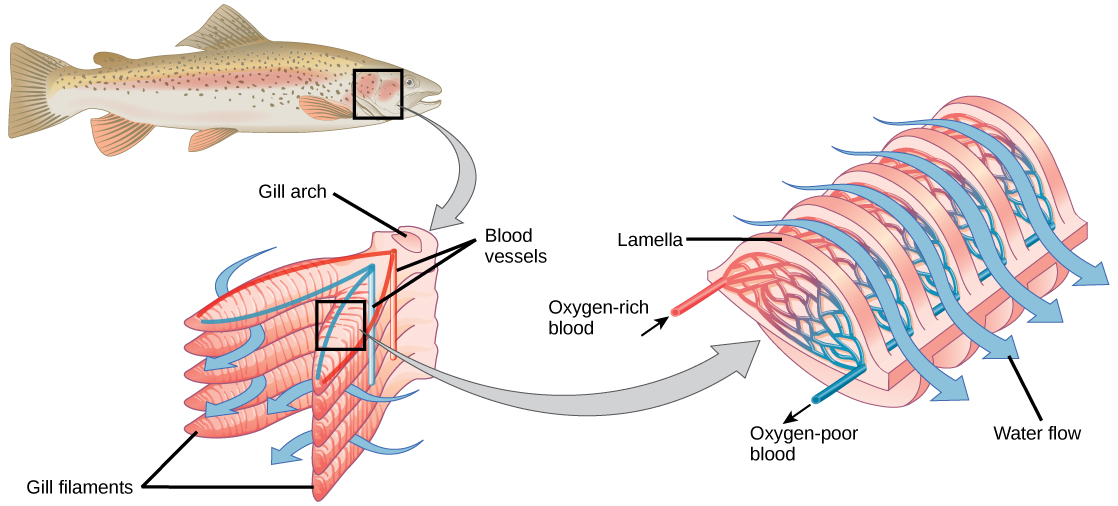
The concentration of oxygen molecules in water is higher than the concentration of oxygen molecules in gills. As a result, oxygen molecules diffuse from water (high concentration) to blood (low concentration), as shown in. Similarly, carbon dioxide molecules in the blood diffuse from the blood (high concentration) to water (low concentration). It will probably help to watch this video that shows how this countercurrent gas exchange works.
Watch this video to see how countercurrent gas exchange is achieved in fish gills: https://youtu.be/cVFqME-NW9s
Quick Review: Countercurrent gas exchange
Word bank: lower, higher, diffuse from, diffuse to, osmose
Tracheal Systems
Insect respiration is independent of its circulatory system; therefore, the blood does not play a direct role in oxygen transport. Insects have a highly specialized type of respiratory system called the tracheal system, which consists of a network of small tubes that carries oxygen to the entire body. The tracheal system is the most direct and efficient respiratory system in active animals. The tubes in the tracheal system are made of a polymeric material called chitin.
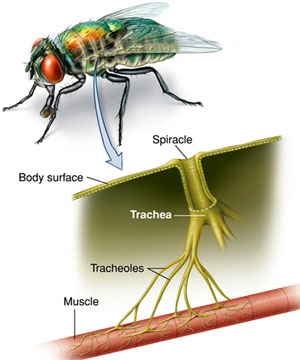
Insect bodies have openings, called spiracles, along the thorax and abdomen. These openings connect to the tubular network, allowing oxygen to pass into the body and regulating the diffusion of CO2 and water vapor. Air enters and leaves the tracheal system through the spiracles. Some insects can ventilate the tracheal system with body movements.
Review Question:
Quick Review: Tracheal Systems
Mammalian Systems
In mammals, pulmonary ventilation occurs via inhalation (breathing). During inhalation, air enters the body through the nasal cavity located just inside the nose. As air passes through the nasal cavity, the air is warmed to body temperature and humidified. The respiratory tract is coated with mucus to seal the tissues from direct contact with air. Mucus is high in water. As air crosses these surfaces of the mucous membranes, it picks up water. These processes help equilibrate the air to the body conditions, reducing any damage that cold, dry air can cause. Particulate matter that is floating in the air is removed in the nasal passages via mucus and cilia. The processes of warming, humidifying, and removing particles are important protective mechanisms that prevent damage to the trachea and lungs. Thus, inhalation serves several purposes in addition to bringing oxygen into the respiratory system.
From the nasal cavity, air passes through the pharynx (throat) and the larynx (voice box), as it makes its way to the trachea. The main function of the trachea is to funnel the inhaled air to the lungs and the exhaled air back out of the body. The human trachea is a cylinder about 10 to 12 cm long and 2 cm in diameter that sits in front of the esophagus and extends from the larynx into the chest cavity where it divides into the two primary bronchi at the midthorax. It is made of incomplete rings of hyaline cartilage and smooth muscle). The trachea is lined with mucus-producing goblet cells and ciliated epithelia. The cilia propel foreign particles trapped in the mucus toward the pharynx. The cartilage provides strength and support to the trachea to keep the passage open. The smooth muscle can contract, decreasing the trachea’s diameter, which causes expired air to rush upwards from the lungs at a great force. The forced exhalation helps expel mucus when we cough. Smooth muscle can contract or relax, depending on stimuli from the external environment or the body’s nervous system.
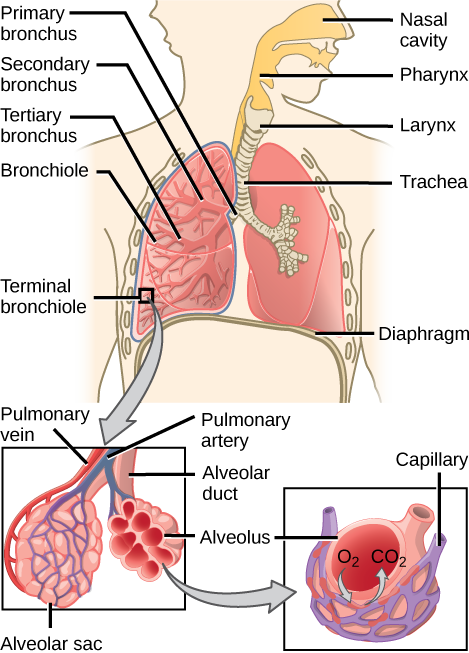
In the lungs, the air is diverted into smaller and smaller passages or bronchi. Air enters the lungs through the two primary (main) bronchi (singular: bronchus). Each bronchus divides into secondary bronchi, then into tertiary bronchi, which in turn divide, creating smaller and smaller diameter bronchioles as they split and spread through the lung. Like the trachea, the bronchi are made of cartilage and smooth muscle. At the bronchioles, the cartilage is replaced with elastic fibers. Bronchi are innervated by nerves of both the parasympathetic and sympathetic nervous systems that control muscle contraction (parasympathetic) or relaxation (sympathetic) in the bronchi and bronchioles, depending on the nervous system’s cues. In humans, bronchioles with a diameter smaller than 0.5 mm are the respiratory bronchioles. They lack cartilage and therefore rely on inhaled air to support their shape. As the passageways decrease in diameter, the relative amount of smooth muscle increases.
The terminal bronchioles subdivide into microscopic branches called respiratory bronchioles. The respiratory bronchioles subdivide into several alveolar ducts. Numerous alveoli and alveolar sacs surround the alveolar ducts. The alveolar sacs resemble bunches of grapes tethered to the end of the bronchioles. In the acinar region, the alveolar ducts are attached to the end of each bronchiole. At the end of each duct are approximately 100 alveolar sacs, each containing 20 to 30 alveoli that are 200 to 300 microns in diameter.
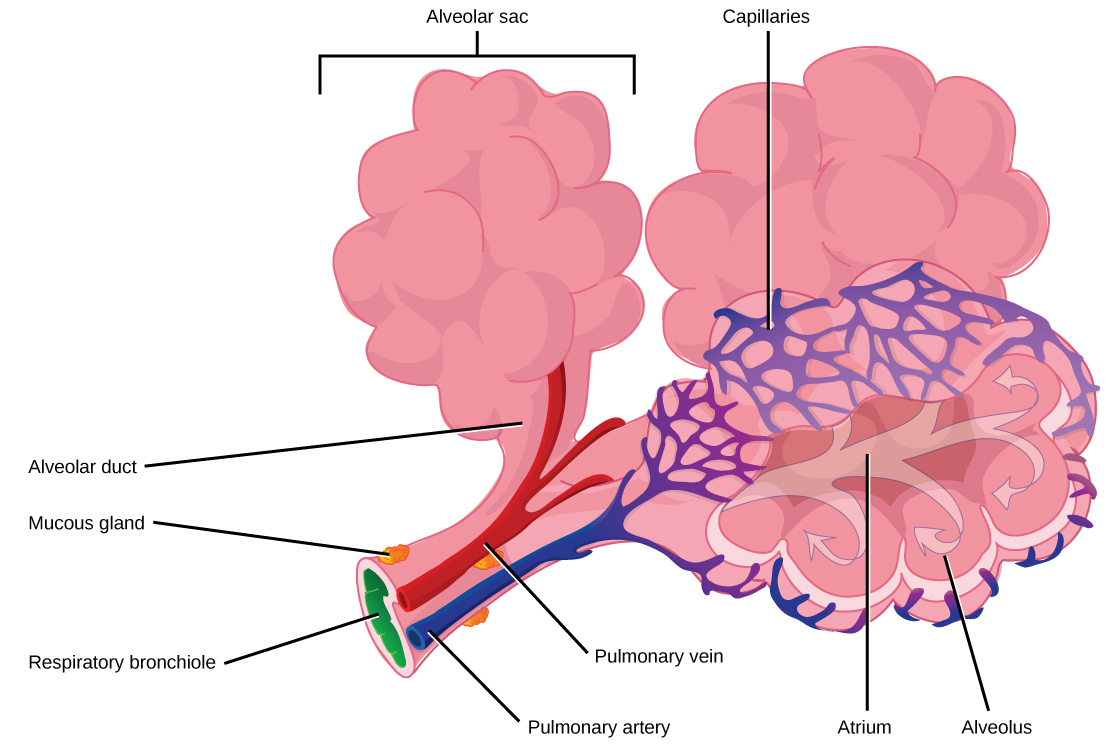
Gas exchange occurs only in alveoli. Alveoli are made of thin-walled parenchymal cells, typically one-cell thick, that look like tiny bubbles within the sacs. Alveoli are in direct contact with capillaries (one-cell thick) of the circulatory system. Such intimate contact ensures that oxygen will diffuse from alveoli into the blood and be distributed to the cells of the body. In addition, the carbon dioxide that was produced by cells as a waste product will diffuse from the blood into alveoli to be exhaled. The anatomical arrangement of capillaries and alveoli emphasizes the structural and functional relationship of the respiratory and circulatory systems. Because there are so many alveoli (~300 million per lung) within each alveolar sac and so many sacs at the end of each alveolar duct, the lungs have a sponge-like consistency. This organization produces a very large surface area that is available for gas exchange. The surface area of alveoli in the lungs is approximately 75 m2. This large surface area, combined with the thin-walled nature of the alveolar parenchymal cells, allows gases to easily diffuse across the cells.
Click here to see the respiratory system in humans in action: https://youtu.be/bfxfydYFlhg
Quick Review: Airflow
Protective Mechanisms
The air that organisms breathe contains particulate matter such as dust, dirt, viral particles, and bacteria that can damage the lungs or trigger allergic immune responses. The respiratory system contains several protective mechanisms to avoid problems or tissue damage. In the nasal cavity, hairs and mucus trap small particles, viruses, bacteria, dust, and dirt to prevent their entry.
If particulates do make it beyond the nose, or enter through the mouth, the bronchi and bronchioles of the lungs also contain several protective devices. The lungs produce mucus—a sticky substance made of mucin, a complex glycoprotein, as well as salts and water—that traps particulates. The bronchi and bronchioles contain cilia, small hair-like projections that line the walls of the bronchi and bronchioles. These cilia beat in unison and move mucus and particles out of the bronchi and bronchioles back up to the throat where it is swallowed and eliminated via the esophagus.
In humans, for example, tar and other substances in cigarette smoke destroy or paralyze the cilia, making the removal of particles more difficult. In addition, smoking causes the lungs to produce more mucus, which the damaged cilia are not able to move. This causes a persistent cough, as the lungs try to rid themselves of particulate matter, and makes smokers more susceptible to respiratory ailments.
Summary
Animal respiratory systems are designed to facilitate gas exchange. In mammals, air is warmed and humidified in the nasal cavity. Air then travels down the pharynx, through the trachea, and into the lungs. In the lungs, air passes through the branching bronchi, reaching the respiratory bronchioles, which house the first site of gas exchange. The respiratory bronchioles open into the alveolar ducts, alveolar sacs, and alveoli. Because there are so many alveoli and alveolar sacs in the lung, the surface area for gas exchange is very large. Several protective mechanisms are in place to prevent damage or infection. These include the hair and mucus in the nasal cavity that trap dust, dirt, and other particulate matter before they can enter the system. In the lungs, particles are trapped in a mucus layer and transported via cilia up to the esophageal opening at the top of the trachea to be swallowed.
Review Questions
REVIEW: Surface area to volume
REVIEW: Gas exchange limitations
3) Why is gas exchange more difficult for aquatic animals with gills than for terrestrial animals with lungs?
Learning Goals:
By the end of this reading you should be able to:
- Describe the different means by which animals exchange gases
- Explain the role that diffusion plays in different systems of gas exchange
- Describe the process of countercurrent gas exchange
- Compare and contrast the tracheal system of insects with the mammalian respiratory system
Introduction
The primary function of the respiratory system is to deliver oxygen to the cells of the body’s tissues and remove carbon dioxide, a cell waste product. All aerobic organisms require oxygen to carry out their metabolic functions. Along the evolutionary tree, different organisms have devised different means of obtaining oxygen from the surrounding atmosphere. As with other organisms, the environment in which the animal lives greatly determines how an animal respires. In addition, the complexity of the respiratory system usually correlates with the size of the organism. As animal size increases, diffusion distances increase and the ratio of surface area to volume drops. In unicellular organisms, diffusion across the cell membrane is sufficient for supplying oxygen to the cell. However, diffusion is a slow, passive transport process. In order for diffusion to be a feasible means of providing oxygen to the cell, the rate of oxygen uptake must match the rate of diffusion across the membrane. In other words, if the cell were very large or thick, diffusion would not be able to provide oxygen quickly enough to the inside of the cell. Therefore, dependence on diffusion as a means of obtaining oxygen and removing carbon dioxide remains feasible only for small organisms or those with highly-flattened bodies, such as many flatworms (Platyhelminthes). Larger organisms had to evolve specialized respiratory tissues, such as gills, lungs, and respiratory passages accompanied by complex circulatory systems, to transport oxygen throughout their entire body.
Direct Diffusion
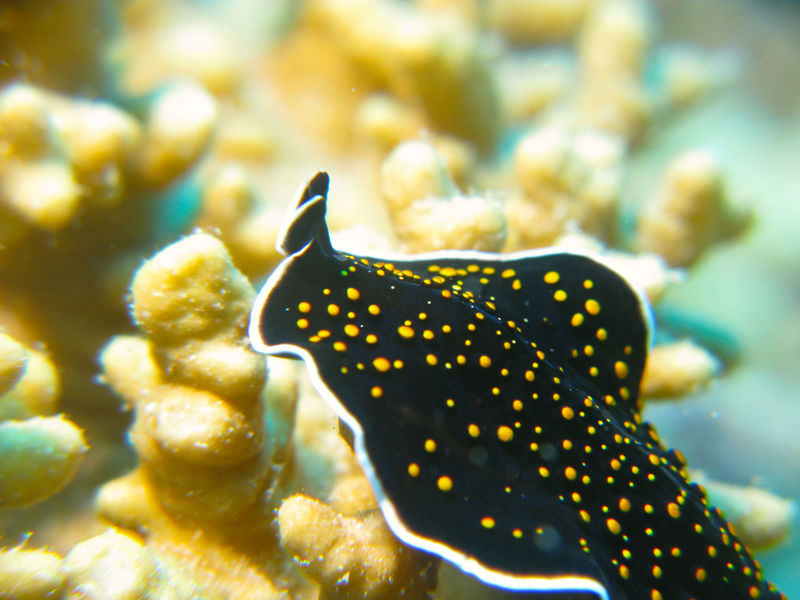
For many small multicellular organisms (less than 1mm in diameter), diffusion across the outer membrane is sufficient to meet their oxygen needs. In simple organisms, such as cnidarians and flatworms (Fig 1), every cell in the body is close to the external environment. Thus the cells are kept moist and gases can diffuse quickly via direct diffusion. Flatworms are small, literally flatworms, which ‘breathe’ through diffusion across the outer membrane. The flat shape of these organisms increases the surface area for diffusion, ensuring that each cell within the body is close to the outer membrane surface and has access to oxygen. If the flatworm had a cylindrical body, then the cells in the center would not be able to get oxygen.
Skin and Gills
Earthworms and amphibians use their skin (integument) as a respiratory organ. A dense network of capillaries lies just below the skin and facilitates gas exchange between the external environment and the circulatory system. The respiratory surface must be kept moist in order for the gases to dissolve and diffuse across cell membranes.
Organisms that live in water need to obtain oxygen from the water. Oxygen dissolves in water but at a lower concentration than in the atmosphere. The atmosphere has roughly 21 percent oxygen. In water, the oxygen concentration is much smaller than that. Fish and many other aquatic organisms have evolved gills to take up the dissolved oxygen from water.
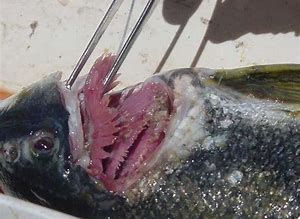
Gills are thin tissue filaments that are highly branched and folded (Fig. 2). When water passes over the gills, the dissolved oxygen in water rapidly diffuses across the gills into the bloodstream. The circulatory system can then carry the oxygenated blood to the other parts of the body. In animals that contain coelomic fluid instead of blood, oxygen diffuses across the gill surfaces into the coelomic fluid. Gills are found in mollusks, annelids, and crustaceans.
The folded surfaces of the gills provide a large surface area to ensure that the fish gets sufficient oxygen. Diffusion is a process in which material travels from regions of high concentration to low concentration until equilibrium is reached. In this case, blood with a low concentration of oxygen molecules circulates through the gills.
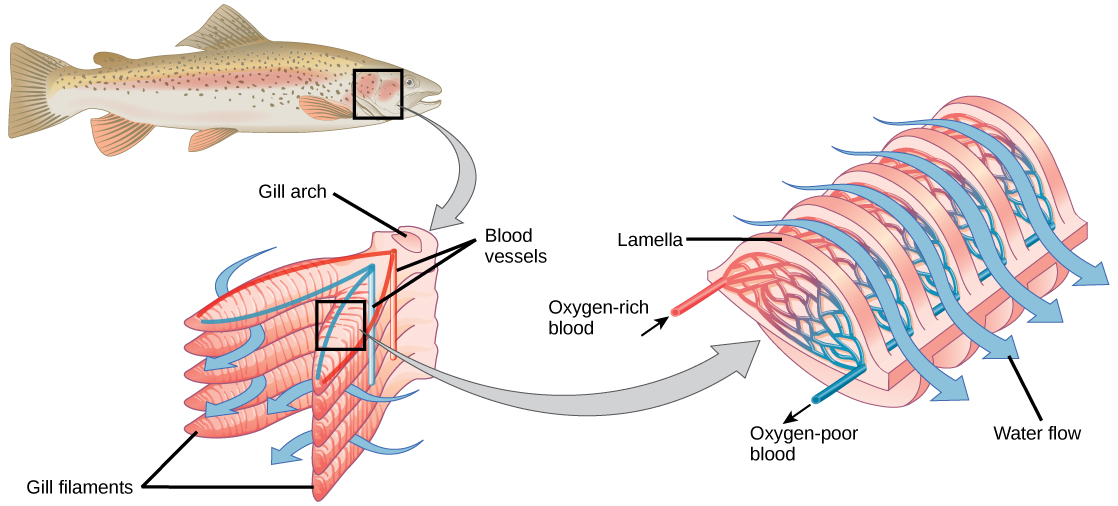
The concentration of oxygen molecules in water is higher than the concentration of oxygen molecules in gills. As a result, oxygen molecules diffuse from water (high concentration) to blood (low concentration), as shown in. Similarly, carbon dioxide molecules in the blood diffuse from the blood (high concentration) to water (low concentration). It will probably help to watch this video that shows how this countercurrent gas exchange works.
Watch this video to see how countercurrent gas exchange is achieved in fish gills: https://youtu.be/cVFqME-NW9s
Quick Review: Countercurrent gas exchange
Word bank: lower, higher, diffuse from, diffuse to, osmose
Tracheal Systems
Insect respiration is independent of its circulatory system; therefore, the blood does not play a direct role in oxygen transport. Insects have a highly specialized type of respiratory system called the tracheal system, which consists of a network of small tubes that carries oxygen to the entire body. The tracheal system is the most direct and efficient respiratory system in active animals. The tubes in the tracheal system are made of a polymeric material called chitin.
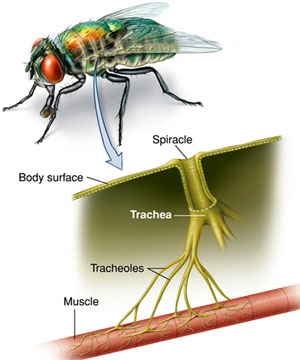
Insect bodies have openings, called spiracles, along the thorax and abdomen. These openings connect to the tubular network, allowing oxygen to pass into the body and regulating the diffusion of CO2 and water vapor. Air enters and leaves the tracheal system through the spiracles. Some insects can ventilate the tracheal system with body movements.
Review Question:
Quick Review: Tracheal Systems
Mammalian Systems
In mammals, pulmonary ventilation occurs via inhalation (breathing). During inhalation, air enters the body through the nasal cavity located just inside the nose. As air passes through the nasal cavity, the air is warmed to body temperature and humidified. The respiratory tract is coated with mucus to seal the tissues from direct contact with air. Mucus is high in water. As air crosses these surfaces of the mucous membranes, it picks up water. These processes help equilibrate the air to the body conditions, reducing any damage that cold, dry air can cause. Particulate matter that is floating in the air is removed in the nasal passages via mucus and cilia. The processes of warming, humidifying, and removing particles are important protective mechanisms that prevent damage to the trachea and lungs. Thus, inhalation serves several purposes in addition to bringing oxygen into the respiratory system.
From the nasal cavity, air passes through the pharynx (throat) and the larynx (voice box), as it makes its way to the trachea. The main function of the trachea is to funnel the inhaled air to the lungs and the exhaled air back out of the body. The human trachea is a cylinder about 10 to 12 cm long and 2 cm in diameter that sits in front of the esophagus and extends from the larynx into the chest cavity where it divides into the two primary bronchi at the midthorax. It is made of incomplete rings of hyaline cartilage and smooth muscle). The trachea is lined with mucus-producing goblet cells and ciliated epithelia. The cilia propel foreign particles trapped in the mucus toward the pharynx. The cartilage provides strength and support to the trachea to keep the passage open. The smooth muscle can contract, decreasing the trachea’s diameter, which causes expired air to rush upwards from the lungs at a great force. The forced exhalation helps expel mucus when we cough. Smooth muscle can contract or relax, depending on stimuli from the external environment or the body’s nervous system.
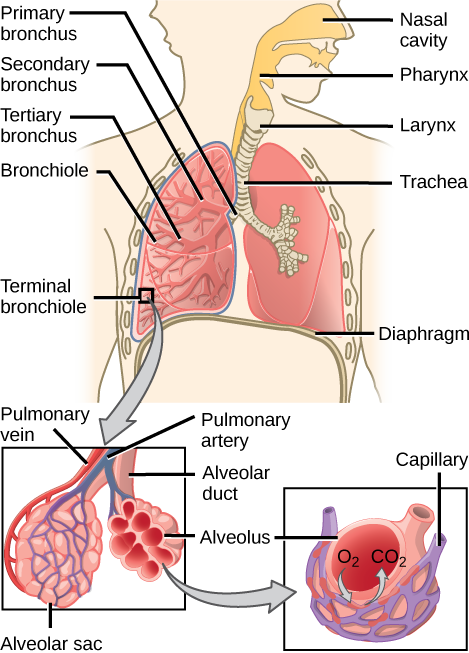
In the lungs, the air is diverted into smaller and smaller passages or bronchi. Air enters the lungs through the two primary (main) bronchi (singular: bronchus). Each bronchus divides into secondary bronchi, then into tertiary bronchi, which in turn divide, creating smaller and smaller diameter bronchioles as they split and spread through the lung. Like the trachea, the bronchi are made of cartilage and smooth muscle. At the bronchioles, the cartilage is replaced with elastic fibers. Bronchi are innervated by nerves of both the parasympathetic and sympathetic nervous systems that control muscle contraction (parasympathetic) or relaxation (sympathetic) in the bronchi and bronchioles, depending on the nervous system’s cues. In humans, bronchioles with a diameter smaller than 0.5 mm are the respiratory bronchioles. They lack cartilage and therefore rely on inhaled air to support their shape. As the passageways decrease in diameter, the relative amount of smooth muscle increases.
The terminal bronchioles subdivide into microscopic branches called respiratory bronchioles. The respiratory bronchioles subdivide into several alveolar ducts. Numerous alveoli and alveolar sacs surround the alveolar ducts. The alveolar sacs resemble bunches of grapes tethered to the end of the bronchioles. In the acinar region, the alveolar ducts are attached to the end of each bronchiole. At the end of each duct are approximately 100 alveolar sacs, each containing 20 to 30 alveoli that are 200 to 300 microns in diameter.
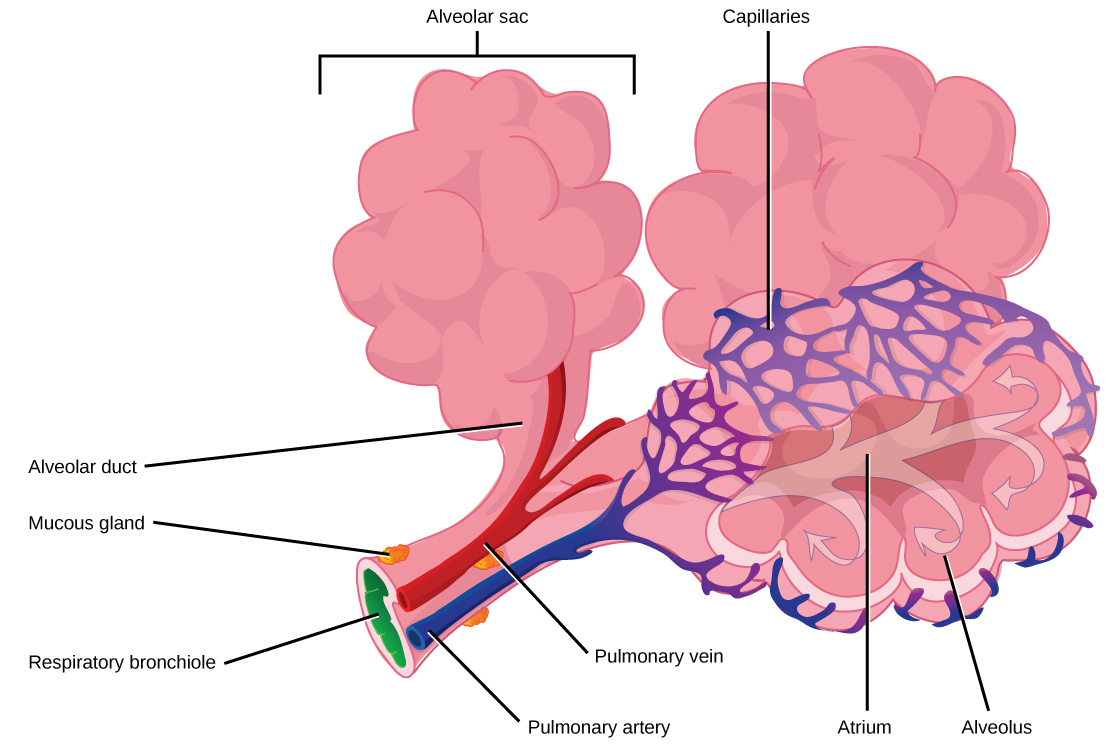
Gas exchange occurs only in alveoli. Alveoli are made of thin-walled parenchymal cells, typically one-cell thick, that look like tiny bubbles within the sacs. Alveoli are in direct contact with capillaries (one-cell thick) of the circulatory system. Such intimate contact ensures that oxygen will diffuse from alveoli into the blood and be distributed to the cells of the body. In addition, the carbon dioxide that was produced by cells as a waste product will diffuse from the blood into alveoli to be exhaled. The anatomical arrangement of capillaries and alveoli emphasizes the structural and functional relationship of the respiratory and circulatory systems. Because there are so many alveoli (~300 million per lung) within each alveolar sac and so many sacs at the end of each alveolar duct, the lungs have a sponge-like consistency. This organization produces a very large surface area that is available for gas exchange. The surface area of alveoli in the lungs is approximately 75 m2. This large surface area, combined with the thin-walled nature of the alveolar parenchymal cells, allows gases to easily diffuse across the cells.
Click here to see the respiratory system in humans in action: https://youtu.be/bfxfydYFlhg
Quick Review: Airflow
Protective Mechanisms
The air that organisms breathe contains particulate matter such as dust, dirt, viral particles, and bacteria that can damage the lungs or trigger allergic immune responses. The respiratory system contains several protective mechanisms to avoid problems or tissue damage. In the nasal cavity, hairs and mucus trap small particles, viruses, bacteria, dust, and dirt to prevent their entry.
If particulates do make it beyond the nose, or enter through the mouth, the bronchi and bronchioles of the lungs also contain several protective devices. The lungs produce mucus—a sticky substance made of mucin, a complex glycoprotein, as well as salts and water—that traps particulates. The bronchi and bronchioles contain cilia, small hair-like projections that line the walls of the bronchi and bronchioles. These cilia beat in unison and move mucus and particles out of the bronchi and bronchioles back up to the throat where it is swallowed and eliminated via the esophagus.
In humans, for example, tar and other substances in cigarette smoke destroy or paralyze the cilia, making the removal of particles more difficult. In addition, smoking causes the lungs to produce more mucus, which the damaged cilia are not able to move. This causes a persistent cough, as the lungs try to rid themselves of particulate matter, and makes smokers more susceptible to respiratory ailments.
Summary
Animal respiratory systems are designed to facilitate gas exchange. In mammals, air is warmed and humidified in the nasal cavity. Air then travels down the pharynx, through the trachea, and into the lungs. In the lungs, air passes through the branching bronchi, reaching the respiratory bronchioles, which house the first site of gas exchange. The respiratory bronchioles open into the alveolar ducts, alveolar sacs, and alveoli. Because there are so many alveoli and alveolar sacs in the lung, the surface area for gas exchange is very large. Several protective mechanisms are in place to prevent damage or infection. These include the hair and mucus in the nasal cavity that trap dust, dirt, and other particulate matter before they can enter the system. In the lungs, particles are trapped in a mucus layer and transported via cilia up to the esophageal opening at the top of the trachea to be swallowed.
Review Questions
REVIEW: Surface area to volume
REVIEW: Gas exchange limitations
3) Why is gas exchange more difficult for aquatic animals with gills than for terrestrial animals with lungs?
Learning Goals
By the end of this reading, you should be able to
- Order the events that lead to the current adaptations of terrestrial tetrapods
- Describe key adaptations that allow for support in terrestrial environments
- Explain how changes in vertebral structure lead to the current mobility of terrestrial tetrapods
- Delineate between ancestral and derived traits of tetrapods
Introduction
The word "tetrapod" means "four feet" and includes all species alive today that have four feet — but this group also includes many animals that don't have four feet. That's because the group includes all the organisms (living and extinct) that descended from the last common ancestor of amphibians, reptiles, birds, and mammals. So, for example, the ichthyosaur, an extinct swimming reptile, is a tetrapod even though it did not use its limbs to walk on land. Snakes are also tetrapods even though they do not have limbs. Birds and humans are tetrapods even though they only walk on two legs. All these animals are tetrapods because they descend from the tetrapod ancestor.
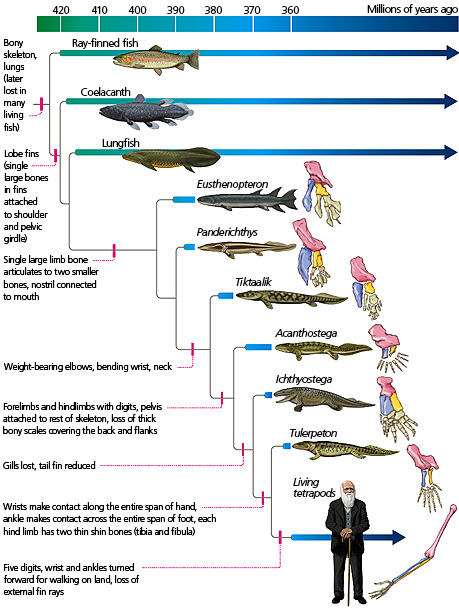
Characteristics of fish ancestors
Most 25,000 living species of animals that we call fishes are ray-finned fishes. There in fact far more of these types of fishes than all the other vertebrates combined. The fins of these fish have a unique structure— that is, they have a system of often branching bony rays (called lepidotrichia) that emanate from the base of the fin. In contrast, the other animals in the phylogenetic tree — coelacanths, lungfishes, all the other extinct animals, plus tetrapods (represented by Charles Darwin) — have what we call "fleshy fins" or "lobe fins." That is, their limbs are covered by muscle and skin. Some, such as coelacanths, retain lepidotrichia at the ends of these fleshy limbs, but in most fleshy-finned animals these have been lost.
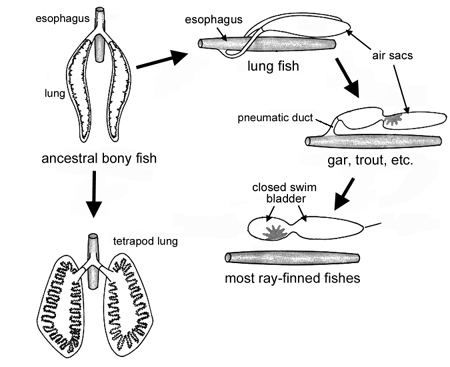
The common ancestor of all those different organisms (ray-fins, coelacanths, lungfishes, tetrapods, etc.) was neither a lobe-fin nor a ray-fin. This ancient vertebrate lineage had fins (with lepidotrichia), scales, gills, and lived in the water. Yet they also had air bladders (air-filled sacs) connected to the back of their throats that could be used for breathing air (i.e., as lungs) or for buoyancy control. The air bladders of many current ray-finned fish no longer connect to their throats, and so they are not able to breathe air. In these ray-fins, the air bladder is used mainly for buoyancy control and is known as a swim bladder. By contrast, tetrapods have taken an alternative route: they have lost the buoyancy control function of their air bladders, and instead, this organ has been altered to form the lungs that we all rely on to provide gases on land.
When we get past coelacanths and lungfishes on the phylogenetic tree, we find a series of fossil forms that lived between about 390 and 360 million years ago during the Devonian Period. During this interval, this lineage of fleshy-finned organisms evolved adaptations that allowed them to leave the water and begin to live on land. Many of these adaptations involved parts of the skeleton that were altered to both structural support and locomotion in a terrestrial environment.
Review Question:
Changes to the skeletons
The ancestors of the tetrapods lived fully in the water and had skulls that were tall and narrow, with eyes facing sideways and forwards. This allowed them to look around in their watery environments for predators and prey. However, as ancestors of the first tetrapods began to live in shallower waters, their skulls evolved to be flatter, with eyes on the tops of their heads. This probably allowed them to look up to spot food. Then, as tetrapods finally moved fully onto land and away from the water, many lineages once again evolved skulls that were tall and narrow, with eyes facing sideways and forwards, allowing them to look around their terrestrial environments for predators and prey.
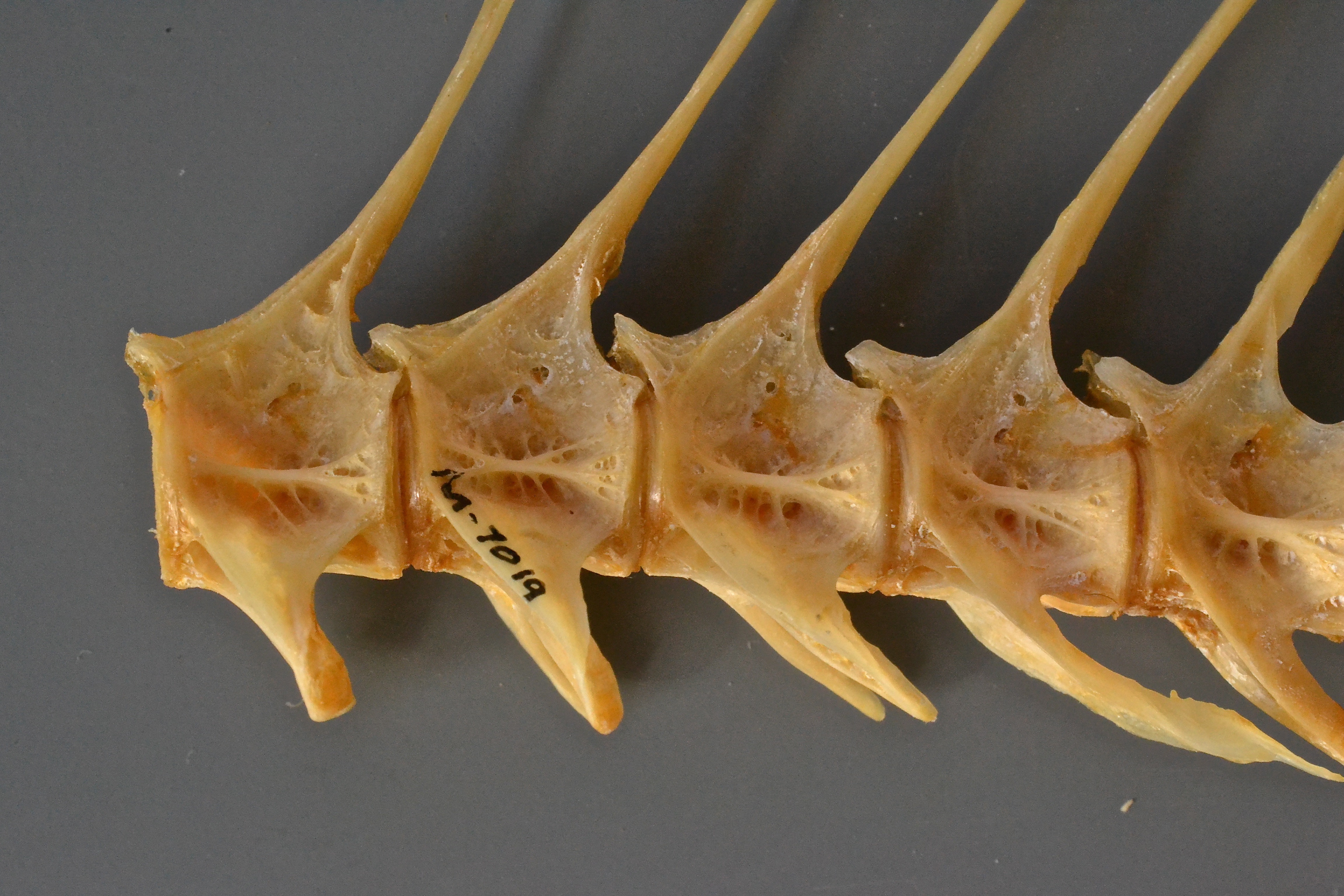
As lineages moved into shallower water and onto land, the vertebral column gradually evolved as well. You may have noticed that fishes have no necks. Their heads are simply connected to their shoulders, and their individual vertebrae look quite similar to one another, all the way down the body. In shallow water dwellers and land dwellers, the first neck vertebra evolved different shapes. This adaptation allowed greater movement of the vertebrae and thus made it possible for these animals to move their heads up and down. Eventually, a second modification of the neck vertebra occurred that made it possible for a group of these animals to move their heads left and right. Mobile necks allow land animals to look down to see the things on the ground that they might want to eat and to watch for predators. Later tetrapods evolved necks with seven or more vertebrae, some long and some short, permitting even more mobility.
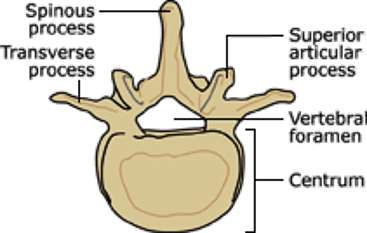
The vertebrae you are probably most familiar with (like our own!) consist of a spool-like centrum, which connects in front and back with other centra. On top of the centra are vertebral spines and arches to which muscle segments attach, and lateral to the centra are the ribs; these anchor muscles that flex as the animals move. Fishes swim with simple lateral motions, so their arches are relatively straight and needle-like, and so are their ribs. When you eat fish and pick out the bones, these are mostly what you're finding. Because fishes live in the water, gravity is not a big problem for them. But on land, a quadruped with a backbone between forelimbs and hindlimbs faces the same problems as a bridge designer: sag. As the fleshy-finned organisms began to venture onto land, they evolved a series of interlocking articulations on each vertebra, which helped them overcome sag and hold the backbone straight with minimal muscular effort.
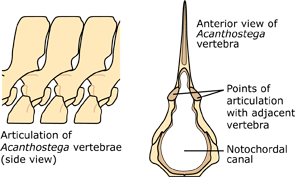
The connection between the pelvis and hindlimbs in early tetrapods is a prime example of exaptation. We call this fused connection the sacrum. It is extremely useful for terrestrial organisms because it allows them to use their hindlimbs efficiently for locomotion on land. Since the aquatic ancestors of fishes and tetrapods had no such connection, one might guess that this feature first evolved serving the function of enabling terrestrial locomotion. However, the earliest form of this connection (as seen in Acanthostega) evolved while these tetrapod precursors were still living in the water. Based on current evidence, Acanthostega appears to have been fully aquatic, so this connection likely evolved to function in something other than terrestrial locomotion. Only later, as tetrapod ancestors moved onto land, was this trait co-opted for terrestrial support — and as it was, additional vertebrae were fused in the same way, providing further support.
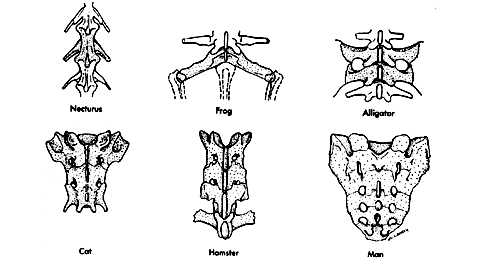
As the limbs and their connections to the rest of the skeleton evolved, limb bones took on distinct roles and many bones were lost. The humerus and the femur were already connected to two outer bones (the radius and ulna in the forelimb, the tibia and fibula in the hindlimb). This is something that evolved about 30 million years before vertebrates came onto land. However, muscular connections between these bones began to change on the road to land and allowed the limbs to be used for terrestrial locomotion. The ankle was originally composed of many small bones arranged in two rows, but gradually many of these small bones were lost. The first animals to get close to walking on land had eight digits on each limb. Over time, some of these digits were lost, leading to animals with seven digits, then six, and then five, which is the common condition now seen in living tetrapods.
Review Question:
Other adaptations to land
As these animals evolved to live on land, other changes in the rest of their bodies evolved. Many would eventually lose their gills, which only work well for getting oxygen when wet, and their tail fins got smaller. Similarly, they lost the lateral line system, a network of vibration-sensitive canals along the skull and jaw, which doesn't work out of water.
The environments of the animals also changed through time. In fact, if you were to venture back to Arizona at the beginning of the "Age of Dinosaurs" in the Triassic Period, some 225 million years ago, you would find ray-fins, coelacanths, and lungfishes living in the marshes, streams, and temporary ponds of that day, along with freshwater sharks. So the habitats that these animals occupy today are not necessarily the ones in which they have always lived, or in which they originally evolved. It is still unclear exactly where the transition from water to land took place ecologically. Paleontologists have discovered fossils involved in this transition preserved from freshwater, brackish, and marine habitats. Regardless of where the transition occurred, eventually, early ancestors of the first tetrapods came up onto land — although not all stayed. Some, like the whales, made the transition back into the water.
Click here to learn more about the origin of the four-legged vertebrates: https://youtu.be/zK8XGEDcTfo
Summary
All modern-day tetrapods share a common ancestry that begins with the fish. It is likely that some ancestral fishes evolved the capacity to breathe air and to move out of the water. The terrestrial environment required changes in the structures of the bones that provided support as well as those involved in the mobility of the organism. Additional adaptations that altered bone morphology and physiological processes occurred as new environments on land were entered.
End of Section Review Questions:
Thinking About It: Changes in bone shapes
Thinking about it: Changes to bone numbers
References:
Learning Goals
By the end of this reading, you should be able to
- Order the events that lead to the current adaptations of terrestrial tetrapods
- Describe key adaptations that allow for support in terrestrial environments
- Explain how changes in vertebral structure lead to the current mobility of terrestrial tetrapods
- Delineate between ancestral and derived traits of tetrapods
Introduction
The word "tetrapod" means "four feet" and includes all species alive today that have four feet — but this group also includes many animals that don't have four feet. That's because the group includes all the organisms (living and extinct) that descended from the last common ancestor of amphibians, reptiles, birds, and mammals. So, for example, the ichthyosaur, an extinct swimming reptile, is a tetrapod even though it did not use its limbs to walk on land. Snakes are also tetrapods even though they do not have limbs. Birds and humans are tetrapods even though they only walk on two legs. All these animals are tetrapods because they descend from the tetrapod ancestor.
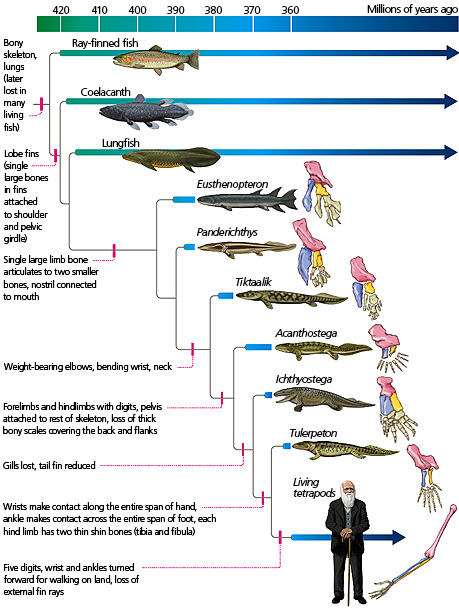
Characteristics of fish ancestors
Most 25,000 living species of animals that we call fishes are ray-finned fishes. There in fact far more of these types of fishes than all the other vertebrates combined. The fins of these fish have a unique structure— that is, they have a system of often branching bony rays (called lepidotrichia) that emanate from the base of the fin. In contrast, the other animals in the phylogenetic tree — coelacanths, lungfishes, all the other extinct animals, plus tetrapods (represented by Charles Darwin) — have what we call "fleshy fins" or "lobe fins." That is, their limbs are covered by muscle and skin. Some, such as coelacanths, retain lepidotrichia at the ends of these fleshy limbs, but in most fleshy-finned animals these have been lost.
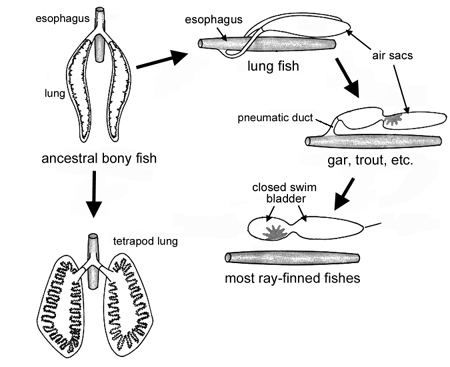
The common ancestor of all those different organisms (ray-fins, coelacanths, lungfishes, tetrapods, etc.) was neither a lobe-fin nor a ray-fin. This ancient vertebrate lineage had fins (with lepidotrichia), scales, gills, and lived in the water. Yet they also had air bladders (air-filled sacs) connected to the back of their throats that could be used for breathing air (i.e., as lungs) or for buoyancy control. The air bladders of many current ray-finned fish no longer connect to their throats, and so they are not able to breathe air. In these ray-fins, the air bladder is used mainly for buoyancy control and is known as a swim bladder. By contrast, tetrapods have taken an alternative route: they have lost the buoyancy control function of their air bladders, and instead, this organ has been altered to form the lungs that we all rely on to provide gases on land.
When we get past coelacanths and lungfishes on the phylogenetic tree, we find a series of fossil forms that lived between about 390 and 360 million years ago during the Devonian Period. During this interval, this lineage of fleshy-finned organisms evolved adaptations that allowed them to leave the water and begin to live on land. Many of these adaptations involved parts of the skeleton that were altered to both structural support and locomotion in a terrestrial environment.
Review Question:
Changes to the skeletons
The ancestors of the tetrapods lived fully in the water and had skulls that were tall and narrow, with eyes facing sideways and forwards. This allowed them to look around in their watery environments for predators and prey. However, as ancestors of the first tetrapods began to live in shallower waters, their skulls evolved to be flatter, with eyes on the tops of their heads. This probably allowed them to look up to spot food. Then, as tetrapods finally moved fully onto land and away from the water, many lineages once again evolved skulls that were tall and narrow, with eyes facing sideways and forwards, allowing them to look around their terrestrial environments for predators and prey.
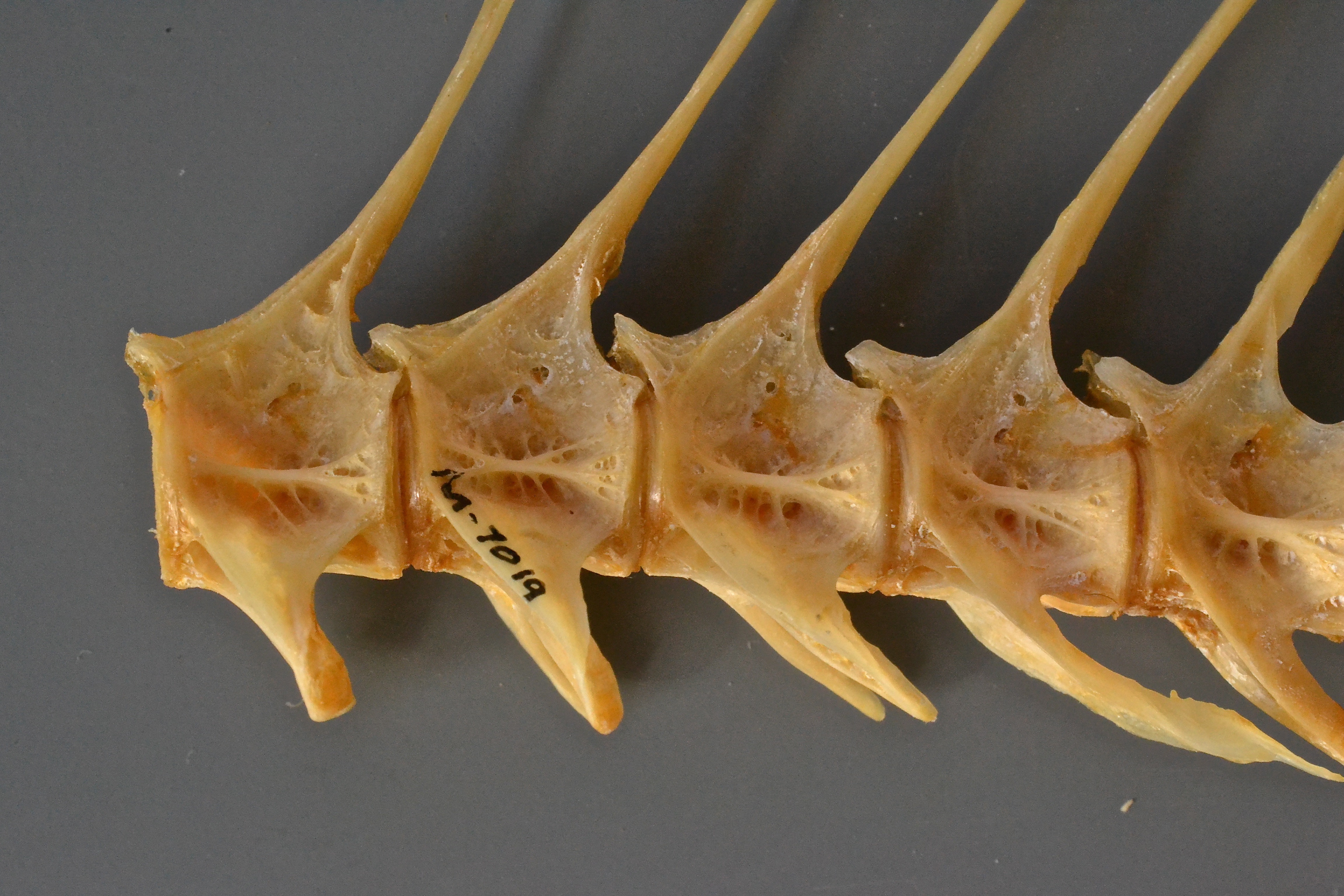
As lineages moved into shallower water and onto land, the vertebral column gradually evolved as well. You may have noticed that fishes have no necks. Their heads are simply connected to their shoulders, and their individual vertebrae look quite similar to one another, all the way down the body. In shallow water dwellers and land dwellers, the first neck vertebra evolved different shapes. This adaptation allowed greater movement of the vertebrae and thus made it possible for these animals to move their heads up and down. Eventually, a second modification of the neck vertebra occurred that made it possible for a group of these animals to move their heads left and right. Mobile necks allow land animals to look down to see the things on the ground that they might want to eat and to watch for predators. Later tetrapods evolved necks with seven or more vertebrae, some long and some short, permitting even more mobility.
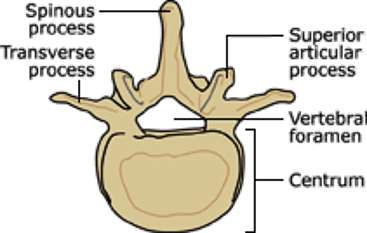
The vertebrae you are probably most familiar with (like our own!) consist of a spool-like centrum, which connects in front and back with other centra. On top of the centra are vertebral spines and arches to which muscle segments attach, and lateral to the centra are the ribs; these anchor muscles that flex as the animals move. Fishes swim with simple lateral motions, so their arches are relatively straight and needle-like, and so are their ribs. When you eat fish and pick out the bones, these are mostly what you're finding. Because fishes live in the water, gravity is not a big problem for them. But on land, a quadruped with a backbone between forelimbs and hindlimbs faces the same problems as a bridge designer: sag. As the fleshy-finned organisms began to venture onto land, they evolved a series of interlocking articulations on each vertebra, which helped them overcome sag and hold the backbone straight with minimal muscular effort.
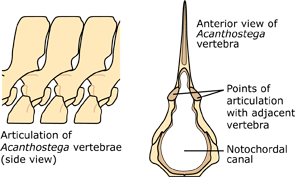
The connection between the pelvis and hindlimbs in early tetrapods is a prime example of exaptation. We call this fused connection the sacrum. It is extremely useful for terrestrial organisms because it allows them to use their hindlimbs efficiently for locomotion on land. Since the aquatic ancestors of fishes and tetrapods had no such connection, one might guess that this feature first evolved serving the function of enabling terrestrial locomotion. However, the earliest form of this connection (as seen in Acanthostega) evolved while these tetrapod precursors were still living in the water. Based on current evidence, Acanthostega appears to have been fully aquatic, so this connection likely evolved to function in something other than terrestrial locomotion. Only later, as tetrapod ancestors moved onto land, was this trait co-opted for terrestrial support — and as it was, additional vertebrae were fused in the same way, providing further support.
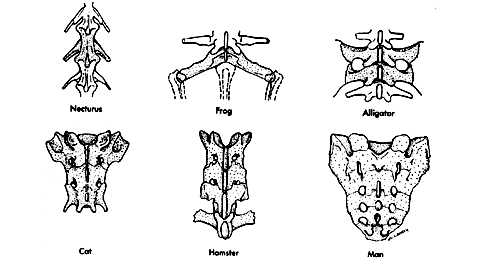
As the limbs and their connections to the rest of the skeleton evolved, limb bones took on distinct roles and many bones were lost. The humerus and the femur were already connected to two outer bones (the radius and ulna in the forelimb, the tibia and fibula in the hindlimb). This is something that evolved about 30 million years before vertebrates came onto land. However, muscular connections between these bones began to change on the road to land and allowed the limbs to be used for terrestrial locomotion. The ankle was originally composed of many small bones arranged in two rows, but gradually many of these small bones were lost. The first animals to get close to walking on land had eight digits on each limb. Over time, some of these digits were lost, leading to animals with seven digits, then six, and then five, which is the common condition now seen in living tetrapods.
Review Question:
Other adaptations to land
As these animals evolved to live on land, other changes in the rest of their bodies evolved. Many would eventually lose their gills, which only work well for getting oxygen when wet, and their tail fins got smaller. Similarly, they lost the lateral line system, a network of vibration-sensitive canals along the skull and jaw, which doesn't work out of water.
The environments of the animals also changed through time. In fact, if you were to venture back to Arizona at the beginning of the "Age of Dinosaurs" in the Triassic Period, some 225 million years ago, you would find ray-fins, coelacanths, and lungfishes living in the marshes, streams, and temporary ponds of that day, along with freshwater sharks. So the habitats that these animals occupy today are not necessarily the ones in which they have always lived, or in which they originally evolved. It is still unclear exactly where the transition from water to land took place ecologically. Paleontologists have discovered fossils involved in this transition preserved from freshwater, brackish, and marine habitats. Regardless of where the transition occurred, eventually, early ancestors of the first tetrapods came up onto land — although not all stayed. Some, like the whales, made the transition back into the water.
Click here to learn more about the origin of the four-legged vertebrates: https://youtu.be/zK8XGEDcTfo
Summary
All modern-day tetrapods share a common ancestry that begins with the fish. It is likely that some ancestral fishes evolved the capacity to breathe air and to move out of the water. The terrestrial environment required changes in the structures of the bones that provided support as well as those involved in the mobility of the organism. Additional adaptations that altered bone morphology and physiological processes occurred as new environments on land were entered.
End of Section Review Questions:
Thinking About It: Changes in bone shapes
Thinking about it: Changes to bone numbers
References:
Learning Goals
By the end of this reading you should be able to:
- Compare and contrast open and closed circulatory systems
- Explain why some animals do not have circulatory systems
- Compare and contrast the organization and evolution of the different vertebrate circulatory systems.
Introduction
The circulatory system is effectively a network of cylindrical vessels: the arteries, veins, and capillaries that emanate from a pump, the heart. In all vertebrate organisms, as well as some invertebrates, this is a closed-loop system, in which the blood is not free in a cavity. In a closed circulatory system, blood is contained inside blood vessels and circulates unidirectionally from the heart around the systemic circulatory route, then returns to the heart again. As opposed to a closed system, arthropods—including insects, crustaceans, and most mollusks—have an open circulatory system. In an open circulatory system, the blood is not enclosed in the blood vessels but is pumped into a cavity called a hemocoel and is called hemolymph because the blood mixes with the interstitial fluid. As the heartbeats and the animal moves, the hemolymph circulates around the organs within the body cavity and then reenters the hearts through openings called ostia. This movement allows for gas and nutrient exchange. An open circulatory system does not use as much energy as a closed system to operate or to maintain; however, there is a trade-off with the amount of blood that can be moved to metabolically active organs and tissues that require high levels of oxygen. In fact, one reason that insects with wingspans of up to two feet wide (70 cm) are not around today is probably because they were outcompeted by the arrival of birds 150 million years ago. Birds, having a closed circulatory system, are thought to have moved more agilely, allowing them to get food faster and possibly to prey on the insects.
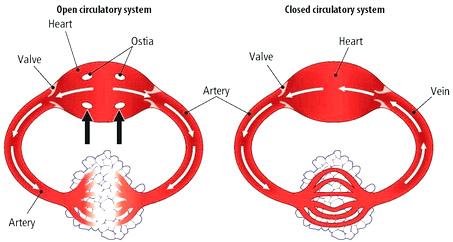
Circulatory System Variation in Animals
The circulatory system varies from simple systems in invertebrates to more complex systems in vertebrates. The simplest animals, such as the sponges (Porifera) and rotifers (Rotifera), do not need a circulatory system because diffusion allows the adequate exchange of water, nutrients, and waste, as well as dissolved gases. Organisms that are more complex but still only have two layers of cells in their body plan, such as jellies (Cnidaria) and comb jellies (Ctenophora) also use diffusion through their epidermis and internally through the gastrovascular compartment. Both their internal and external tissues are bathed in an aqueous environment and exchange fluids by diffusion on both sides. The exchange of fluids is assisted by the pulsing of the jellyfish's body.
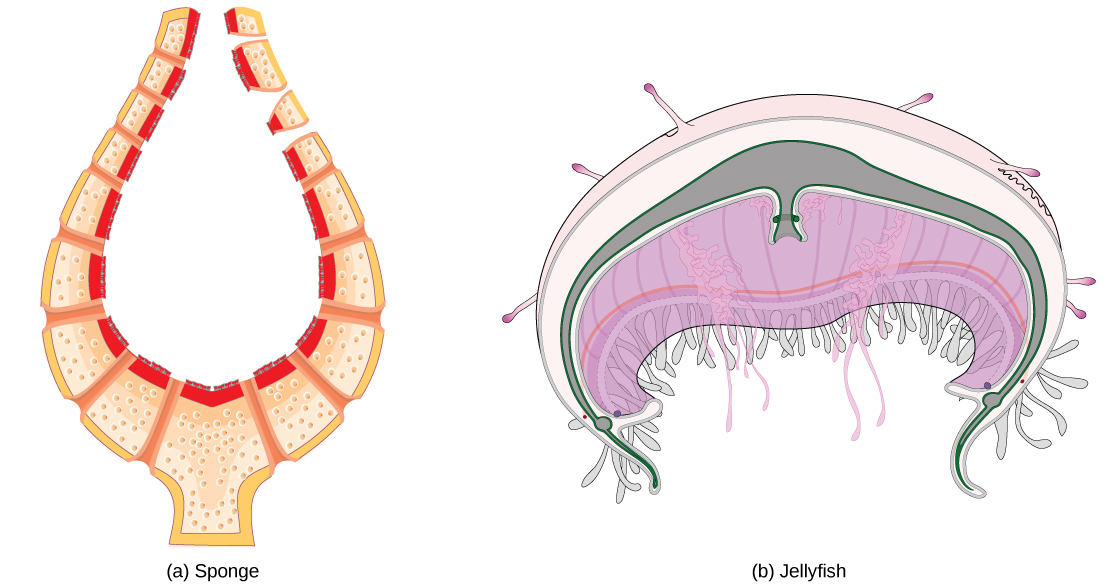
For more complex organisms, diffusion is not efficient for cycling gases, nutrients, and waste effectively through the body; therefore, more complex circulatory systems evolved. Most arthropods and many mollusks have open circulatory systems. In an open system, an elongated beating heart pushes the hemolymph through the body and muscle contractions help to move fluids. The larger more complex crustaceans, including lobsters, have developed arterial-like vessels to push blood through their bodies, and the most active mollusks, such as squids, have evolved a closed circulatory system and are able to move rapidly to catch prey. Closed circulatory systems are a characteristic of vertebrates; however, there are significant differences in the structure of the heart and the circulation of blood between the different vertebrate groups due to adaptation during evolution and associated differences in anatomy.
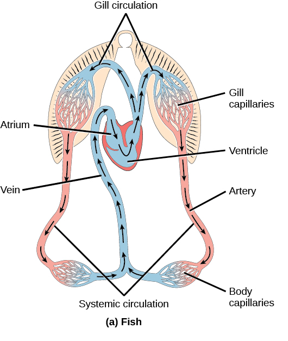
Fish have a single circuit for blood flow and a two-chambered heart that has only a single atrium and a single ventricle. The atrium collects blood that has returned from the body and the ventricle pumps the blood to the gills where gas exchange occurs and the blood is re-oxygenated; this is called gill circulation. The blood then continues through the rest of the body before arriving back at the atrium; this is called systemic circulation. This unidirectional flow of blood produces a gradient of oxygenated to deoxygenated blood around the fish’s systemic circuit. The result is a limit in the amount of oxygen that can reach some of the organs and tissues of the body, reducing the overall metabolic capacity of fish.
In amphibians, reptiles, birds, and mammals, blood flow is directed in two circuits: one through the lungs and back to the heart, which is called pulmonary circulation, and the other throughout the rest of the body and its organs including the brain (systemic circulation). In amphibians, gas exchange also occurs through the skin during pulmonary circulation and is referred to as pulmocutaneous circulation.
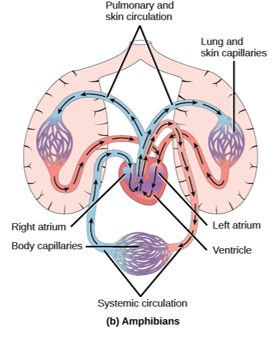
Amphibians have a three-chambered heart that has two atria and one ventricle rather than the two-chambered heart of fish. The two atria (superior heart chambers) receive blood from the two different circuits (the lungs and the systems), and then there is some mixing of the blood in the heart’s ventricle (inferior heart chamber), which reduces the efficiency of oxygenation. The advantage of this arrangement is that high pressure in the vessels pushes blood to the lungs and body. The mixing is mitigated by a ridge within the ventricle that diverts oxygen-rich blood through the systemic circulatory system and deoxygenated blood to the pulmocutaneous circuit. For this reason, amphibians are often described as having double circulation.
Most reptiles also have a three-chambered heart similar to the amphibian heart that directs blood to the pulmonary and systemic circuits. The ventricle is divided more effectively by a partial septum, which results in less mixing of oxygenated and deoxygenated blood.
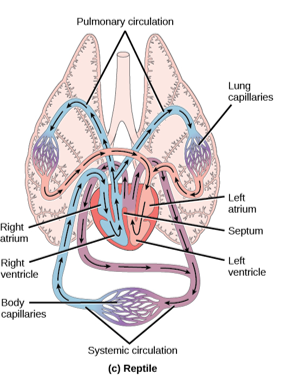
Some reptiles (alligators and crocodiles) are the most primitive animals to exhibit a four-chambered heart. Crocodilians have a unique circulatory mechanism where the heart shunts blood from the lungs toward the stomach and other organs during long periods of submergence, for instance, while the animal waits for prey or stays underwater waiting for prey to rot. One adaptation includes two main arteries that leave the same part of the heart: one takes blood to the lungs and the other provides an alternate route to the stomach and other parts of the body. Two other adaptations include a hole in the heart between the two ventricles, called the foramen of Panizza, which allows blood to move from one side of the heart to the other, and specialized connective tissue that slows the blood flow to the lungs. Together these adaptations have made crocodiles and alligators one of the most evolutionarily successful animal groups on earth.
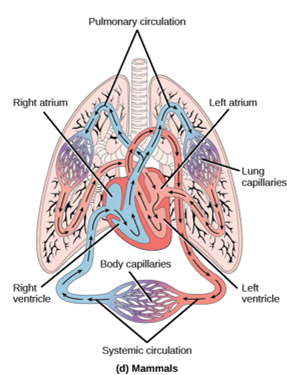
In mammals and birds, the heart is also divided into four chambers: two atria and two ventricles. The oxygenated blood is separated from the deoxygenated blood, which improves the efficiency of double circulation and is probably required for the warm-blooded lifestyle of mammals and birds. The four-chambered heart of birds and mammals evolved independently from a three-chambered heart. The independent evolution of the same or a similar biological trait is referred to as convergent evolution.
Summary
In most animals, the circulatory system is used to transport blood through the body. Some primitive animals use diffusion for the exchange of water, nutrients, and gases. However, complex organisms use the circulatory system to carry gases, nutrients, and waste through the body. Circulatory systems may be open (mixed with the interstitial fluid) or closed (separated from the interstitial fluid). Closed circulatory systems are a characteristic of vertebrates; however, there are significant differences in the structure of the heart and the circulation of blood between the different vertebrate groups due to adaptions during evolution and associated differences in anatomy. Fish have a two-chambered heart with unidirectional circulation. Amphibians have a three-chambered heart, which has some mixing of the blood, and they have double circulation. Most non-avian reptiles have a three-chambered heart, but have little mixing of the blood; they have double circulation. Mammals and birds have a four-chambered heart with no mixing of blood and double circulation.
End of Section Review Questions:
REVIEW: Open circulation
REVIEW: Diffusion
D) sponges
REVIEW: Unique adaptations