6
Learning Goals
-
Describe how natural selection can result in adaptive evolution
-
Differentiate between stabilizing, directional and diversifying selection.
-
Describe how these different forces can lead to different outcomes in terms of the population variation
-
Explain how frequency-dependent selection can impact the genetic structure of a population
-
Describe the roles of sexual selection in the evolution of traits in populations
Introduction
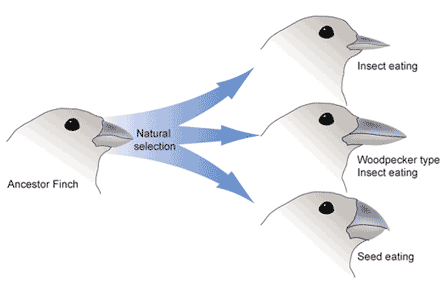
Natural selection only acts on the population’s heritable traits: selecting for beneficial alleles and thus increasing their frequency in the population, while selecting against deleterious alleles and thereby decreasing their frequency—a process known as adaptive evolution (Fig. 1). Natural selection acts on entire organisms though and not on individual alleles. Natural selection acts at the level of the individual; and involves differences in individual contributions to the gene pool of the next generation, known as an organism’s evolutionary (Darwinian) fitness. An individual may carry a very beneficial genotype with a resulting phenotype that, for example, increases the ability to reproduce (fecundity), but if that same individual also carries an allele that results in a fatal childhood disease, that fecundity phenotype will not be passed on to the next generation because the individual will not live to reach reproductive age.
Fitness is often quantifiable and is measured by scientists in the field. However, it is not the absolute fitness of an individual that counts, but rather how it compares to the other organisms in the population. This concept, called relative fitness, allows researchers to determine which individuals are contributing a differential number of offspring to the next generation, and thus, how the population might evolve. As natural selection influences the allele frequencies in a population, individuals can either become more or less genetically similar and as a result, the phenotypes displayed can also become less variable (more similar) or more variable (less similar).
Stabilizing Selection
Directional Selection
Review Question:
Diversifying Selection
Diversifying selection can also occur when environmental changes favor individuals on either end of the phenotypic spectrum (Fig. 4). Imagine a population of mice living at the beach where there is light-colored sand interspersed with patches of tall grass. In this scenario, light-colored mice that blend in with the sand would be favored, as well as dark-colored mice that can hide in the grass. Medium-colored mice, on the other hand, would not blend in with either the grass or the sand, and would thus be more likely to be eaten by predators. The result of this type of selection is increased genetic variance as the population becomes more diverse.
Review Question:
A scientist measures the circumference of acorns in a population of oak trees and discovers that the most common circumference is 2 cm. What would you expect the most common circumference(s) to be after 10 generations of diversifying selection?
A) still 2 cm
Frequency-dependent Selection
In this scenario, orange males will be favored by natural selection when the population is dominated by blue males, blue males will thrive when the population is mostly yellow males, and yellow males will be selected for when orange males are the most populous. As a result, populations of side-blotched lizards cycle in the distribution of these phenotypes—in one generation, orange might be predominant, and then yellow males will begin to rise in frequency. Once yellow males make up a majority of the population, blue males will be selected. Finally, when blue males become common, orange males will once again be favored. Negative frequency-dependent selection serves to increase the population’s genetic variance by selecting for rare phenotypes, whereas positive frequency-dependent selection usually decreases genetic variance by selecting for common phenotypes.
Sexual Selection
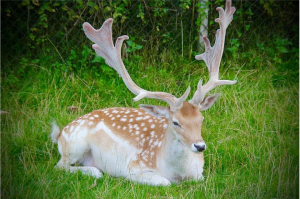
Males and females of certain species are often quite different from one another in ways beyond the reproductive organs. Males are often larger, for example, and display many elaborate colors and adornments, like the peacock’s tail, while females tend to be smaller and duller in decoration. Such differences are known as sexual dimorphisms, which arise from the fact that in many populations, particularly animal populations, there is more variance in the reproductive success of the males than there is of the females. That is, some males—often the bigger, stronger, or more decorated males—get the vast majority of the total mating, while others receive none.
This can occur because the males are better at fighting other males, or because females will choose to mate with the bigger or more decorated males (Fig. 6). In either case, this variation in reproductive success generates a strong selection pressure among males to successfully mate, resulting in the evolution of bigger body size and elaborate ornaments to get the females’ attention. Females, on the other hand, tend to mate less frequently; therefore, they are more likely to select more desirable males each time.
Sexual dimorphism varies widely among species, of course, and some species are even sex-role reversed. In such cases, females tend to have a greater variance in their reproductive success than males and are correspondingly selected for the bigger body size and elaborate traits usually characteristic of males (Fig. 7).
The good genes hypothesis states that males develop these impressive ornaments to show off their efficient metabolism or their ability to fight disease. Females then choose males with the most impressive traits because it signals their genetic superiority, which they will then pass on to their offspring. Though it might be argued that females should not be picky because it will likely reduce their number of offspring, if better males father more fit offspring, it may be beneficial. Fewer, healthier offspring may increase the chances of survival more than many, weaker offspring. In both the handicap principle and the good genes hypothesis, the trait is said to be an honest signal of the males’ quality, thus giving females a way to find the fittest mates— males that will pass the best genes to their offspring.
No Perfect Organism
Furthermore, natural selection can be constrained by the relationships between different polymorphisms. One morph may confer a higher fitness than another, but may not increase in frequency due to the fact that going from the less beneficial to the more beneficial trait would require intermediate morphs that are a less beneficial phenotype. Think back to the mice that live at the beach. Some are light-colored and blend in with the sand, while others are dark and blend in with the patches of grass. The dark-colored mice may be, overall, more fit than the light-colored mice, and at first glance, one might expect that through selection the gradual change to dark coloration would be favored. However, remember that the intermediate phenotype, a medium-colored coat, is not favorable for the mice—they cannot blend in with either the sand or the grass and are more likely to be eaten by predators. As a result, the gradual change to a dark coloration would not occur because those individuals that began displaying an intermediate phenotype would be less fit than those individuals who retained the light coloration.
Finally, it is important to understand that not all evolution is adaptive. While natural selection selects the fittest individuals and often results in a more fit population overall, other forces of evolution, including genetic drift and gene flow, often do the opposite: introducing deleterious alleles to the population’s gene pool. Evolution has no purpose—it is not changing a population into a preconceived ideal. It is simply the sum of the various forces described in this reading and how they influence the genetic and phenotypic variance of a population.
Summary
Because natural selection acts to increase the frequency of beneficial alleles and traits in a given environment while decreasing the frequency of deleterious qualities, it is adaptive evolution. Natural selection acts at the level of the individual, selecting for those that have a higher overall fitness compared to the rest of the population and resulting in differential reproductive success. If the fit phenotypes are those that are similar, natural selection will result in stabilizing selection, and an overall decrease in the population’s variation in those phenotypes will occur. Directional selection works to shift a population’s variance toward a new, fit phenotype, as environmental conditions change. In contrast, diversifying selection results in increased genetic variance by selecting for two or more distinct phenotypes.
Other types of selection include frequency-dependent selection, in which individuals with either common (positive frequency-dependent selection) or rare (negative frequency-dependent selection) are selected for. Finally, sexual selection results from the fact that one sex has more variance in the reproductive success than the other. As a result, males and females experience different selective pressures, which can often lead to the evolution of phenotypic differences, or sexual dimorphisms, between the two.
End of Section Review Questions:
Review: Sexual Selection 1
2) When males and females of a population look or act differently, it is referred to as?
Review: Sexual Selection 2
3) The good genes hypothesis is a theory that explains what?
CRITICAL THINKING QUESTION
Attributions
Footnotes
- [1]Sahar S. Hanania, Dhia S. Hassawi, and Nidal M. Irshaid, ftAllele Frequency and Molecular Genotypes of ABO Blood Group System in a Jordanian Population,ft Journal of Medical Sciences 7 (2007): 51-58, doi:10.3923/jms.2007.51.58.
- [2]A. J. Tipping et al., ftMolecular and Genealogical Evidence for a Founder Efect in Fanconi Anemia Families of the Afrikaner Population of South Africa,ft PNAS98, no. 10 (2001): 5734-5739, doi: 10.1073/pnas.091402398.
AttributionsText: Adapted from OpenStax Biology 2nd Edition, Biology 2e. OpenStax CNX. Nov 26, 2018 http://cnx.org/contents/8d50a0af-948b-4204-a71d-4826cba765b8@15.1.
Figure 1. Image courtesy of National Human Genome Research Institute’s Talking Glossary [Public domain], via Wikimedia Commons
Figure 2. Image modified from OpenStax Biology 2nd Edition, Biology 2e. OpenStax CNX. Nov 26, 2018 http://cnx.org/contents/8d50a0af-948b-4204-a71d-4826cba765b8@15.1
Figure 3. Modified by D. Jennings from image created by Pearson Scott Foresman [Public domain], via Wikimedia Commons
Figure 4. Image modified from OpenStax Biology 2nd Edition, Biology 2e. OpenStax CNX. Nov 26, 2018 http://cnx.org/contents/8d50a0af-948b-4204-a71d-4826cba765b8@15.1
Figure 5. Blue male image and orange male images from the public domain. Combined by D. Jennings
Figure 6. Male deer CC By 0, public domain
Figure 7. Spider: modification of work by ftSanba38ft/Wikimedia Commons; Duck: modification of work by Kevin Cole
Learning Goals
By the end of this reading, you should be able to:
- Discuss the predator-prey cycle
- Give examples of defenses against predation and herbivory
- Describe the competitive exclusion principle
- Give examples of symbiotic relationships between species
- Explain how species interactions can play a role in the adaptation and evolution of species
Introduction
Populations rarely, if ever, live in isolation from populations of other species. In most cases, numerous species share a habitat. The interactions between these populations play a major role in regulating population growth and abundance. All populations occupying the same habitat form a community: populations inhabiting a specific area at the same time. The number of species occupying the same habitat and their relative abundance is known as species diversity. Areas with low diversity, such as the glaciers of Antarctica, still contain a wide variety of living things, whereas the diversity of tropical rainforests is so great that it cannot be counted. Ecology is studied at the community level to understand how species interact with each other and compete for the same resources.
Predation and Herbivory
Perhaps the classical example of species interaction is predation: the consumption of prey by its predator. While we usually think of animals hunting other animals, herbivory, in which plants are eaten by animals is also a form of predation. The predator-prey relationship is considered to be an antagonist one, where one species has a negative impact on the other species. Within a community the sizes of the populations of predators and prey are often not constant over time: in most cases, the respective populations' sizes will vary in cycles that appear to be related.
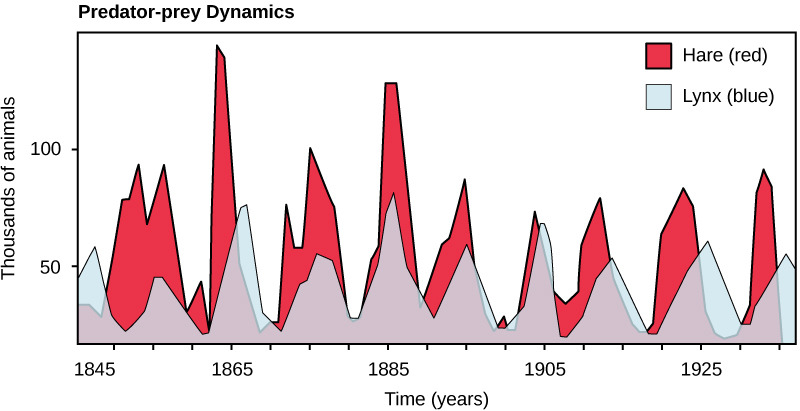
The most often cited example of predator-prey dynamics is seen in the cycling of the lynx (predator) and the snowshoe hare (prey), using nearly 200-year-old trapping data from North American forests. This cycle of predator and prey lasts approximately 10 years, with the predator population lagging 1–2 years behind that of the prey population. As the hare numbers increase, there is more food available for the lynx, allowing the lynx population to increase as well. When the lynx population grows to a threshold level, however, they kill so many hares that the hare population begins to decline, followed by a decline in the lynx population because of the scarcity of food. When the lynx population is low, the hare population size begins to increase due, at least in part, to low predation pressure, starting the cycle anew.
Some researchers question the idea that predation models entirely control the population cycling of the two species. More recent studies have pointed to undefined density-dependent factors as being important in cycling, in addition to predation. One possibility is that the cycling is inherent in the hare population due to density-dependent effects such as lower fecundity (maternal stress) caused by crowding when the hare population gets too dense. The hare cycling would then induce the cycling of the lynx because it is the lynxes’ major food source. The more we study communities, the more complexities we find, allowing ecologists to derive more accurate and sophisticated models of population dynamics.
Review Question:
Defense Mechanisms against Predation and Herbivory
The study of communities must consider evolutionary forces that act on the members of the various populations contained within it. Species are not static, but slowly changing and adapting to their environment by natural selection and other evolutionary forces. Species have evolved numerous mechanisms to escape predation and herbivory. These defenses may be mechanical, chemical, physical, or behavioral.
Mechanical defenses, such as the presence of thorns on plants or the hard shell on turtles, discourage animal predation and herbivory by causing physical pain to the predator or by physically preventing the predator from being able to eat the prey. Chemical defenses are produced by many animals as well as plants, such as the foxglove which is extremely toxic when eaten.
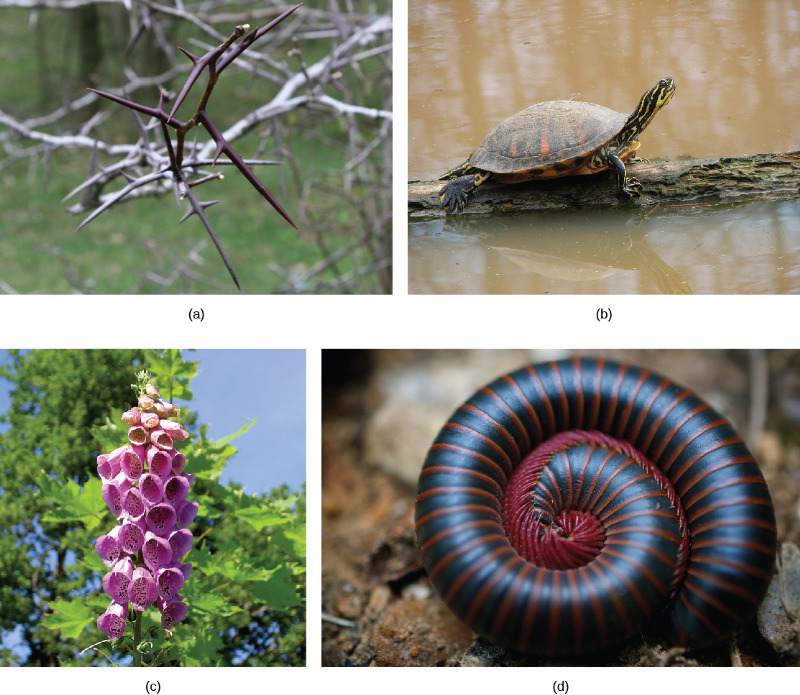
The (a) honey locust tree (Gleditsia triacanthos) uses thorns, a mechanical defense, against herbivores, while the (b) Florida red-bellied turtle (Pseudemys nelsoni) uses its shell as a mechanical defense against predators. (c) Foxglove (Digitalis sp.) uses a chemical defense: toxins produced by the plant can cause nausea, vomiting, hallucinations, convulsions, or death when consumed. (d) The North American millipede (Narceus americanus) uses both mechanical and chemical defenses: when threatened, the millipede curls into a defensive ball and produces a noxious substance that irritates eyes and skin.
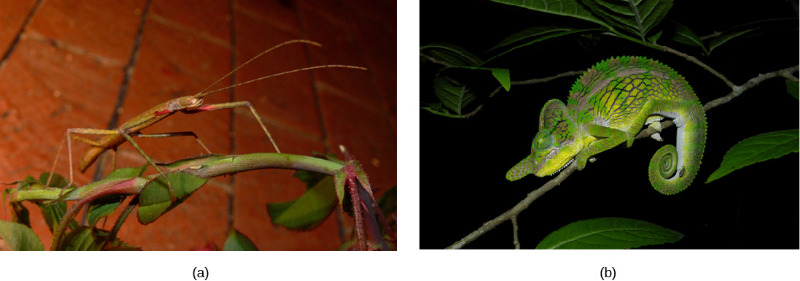
Other species use their body shape and coloration to avoid being detected by predators. The tropical walking stick is an insect with the coloration and body shape of a twig (a) which makes it very hard to see when stationary against a background of real twigs. In another example, the chameleon can change its color to match its surroundings (b). Both of these are examples of camouflage or avoiding detection by blending in with the background.
The use of specific coloration as a way of warning predators to avoid eating them can be found in some species of organisms. For example, the cinnabar moth caterpillar, the fire-bellied toad, and many species of beetle have bright colors that warn of a foul taste, the presence of toxic chemicals, and/or the ability to sting or bite, respectively. Predators that ignore this coloration and eat the organisms will experience their unpleasant taste or presence of toxic chemicals and learn not to eat them in the future. This type of defensive mechanism is called aposematic coloration, or warning coloration.
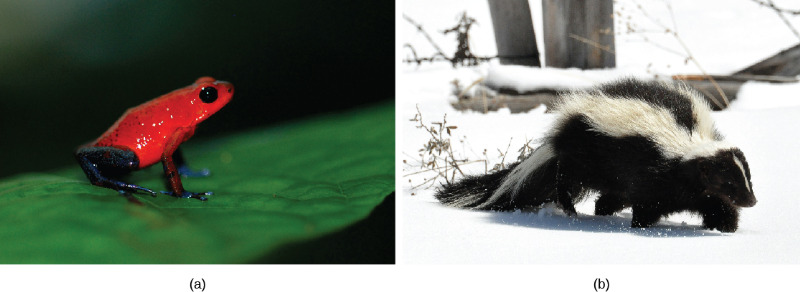
The strawberry poison dart frog (Oophaga pumilio) uses aposematic coloration to warn predators that it is toxic, while the (b) striped skunk (Mephitis mephitis) uses aposematic coloration to warn predators of the unpleasant odor it produces.
While some predators learn to avoid eating certain potential prey because of their coloration, other species have evolved mechanisms to mimic this coloration to avoid being eaten, even though they themselves may not be unpleasant to eat or contain toxic chemicals. In Batesian mimicry, a harmless species imitates the warning coloration of a harmful one. Assuming they share the same predators, this coloration then protects the harmless ones, even though they do not have the same level of physical or chemical defenses against predation as the organism they mimic. Many insect species mimic the coloration of wasps or bees, which are stinging, venomous insects, thereby discouraging predation.
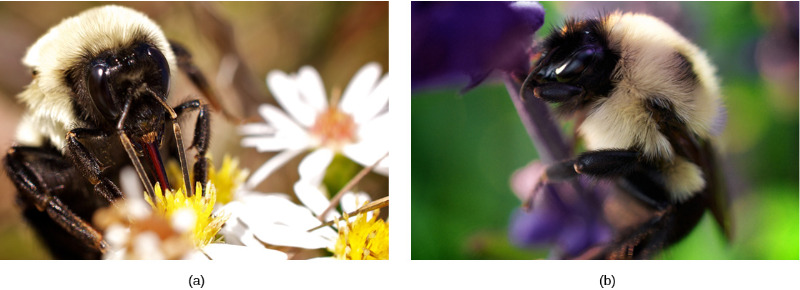
In Müllerian mimicry, multiple species share the same warning coloration, but all of them actually have defenses. This means that they mimic each other in some way creating a larger grouping and thus can be more easily recognized by potential predators.
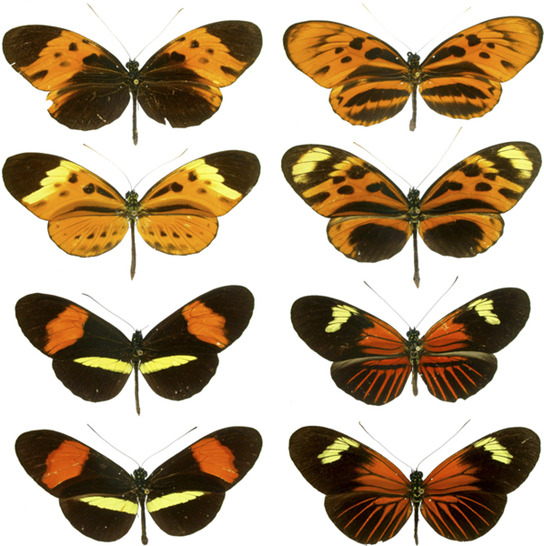
In Emsleyan/Mertensian mimicry, a deadly prey mimics a less dangerous one, such as the venomous coral snake mimicking the nonvenomous milk snake. This type of mimicry is extremely rare and more difficult to understand than the previous two types. For this type of mimicry to work, it is essential that eating the milk snake has unpleasant but not fatal consequences. Then, these predators learn not to eat snakes with this coloration, protecting the coral snake as well. If the snake were fatal to the predator, there would be no opportunity for the predator to learn not to eat it, and the benefit for the less toxic species would disappear.
Review Question:
Quick Review: Mimcry
In mimicry, a toxic species mimics a non-toxic or less toxic species
In a non-toxic/not dangerous species mimics a more toxic/dangerous species
Competitive Exclusion Principle
Resources are often limited within a habitat and multiple species may compete to obtain them. All species have an ecological niche in the ecosystem, which describes how they acquire the resources they need and how they interact with other species in the community. The competitive exclusion principle states that two species cannot occupy the same niche in a habitat. In other words, different species cannot coexist in a community if they are competing for all the same resources. An example of this principle can be seen with two protozoan species, Paramecium aurelia and Paramecium caudatum.
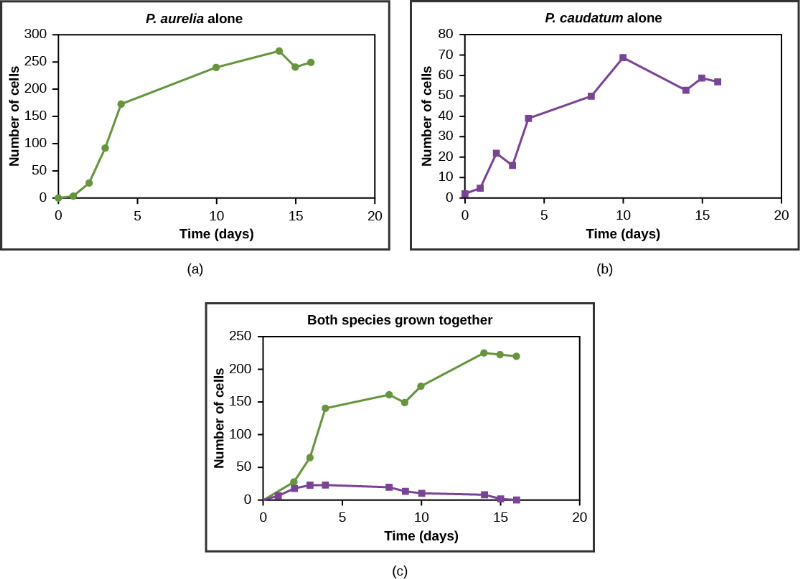
When grown individually in the laboratory, they both thrive. But when they are placed together in the same test tube (habitat), P. aurelia outcompetes P. caudatum for food, leading to the latter’s eventual extinction. This exclusion may be avoided if a population evolves to make use of a different resource, a different area of the habitat, or feeds during a different time of day, called resource partitioning. The two organisms are then said to occupy different microniches. These organisms coexist by minimizing direct competition.
Review Question:
Symbiosis
Symbiotic relationships, or symbioses (plural), are close interactions between individuals of different species over an extended period of time that impact the abundance and distribution of the associating populations. Most scientists accept this definition, but some restrict the term to only those species that are mutualistic, where both individuals benefit from the interaction. In this discussion, the broader definition will be used.
Commensalism
A commensal relationship occurs when one species benefits from the close, prolonged interaction, while the other neither benefits nor is harmed. Birds nesting in trees provides an example of a commensal relationship.
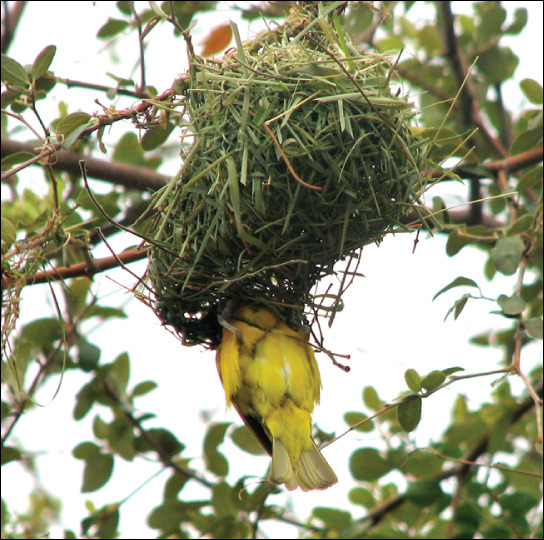
Typically the tree is not harmed by the presence of the nest among its branches. The nests are light and produce little strain on the structural integrity of the branch, and most of the leaves, which the tree uses to get energy by photosynthesis, are above the nest so they are unaffected. The bird, on the other hand, benefits greatly. If the bird had to nest in the open, its eggs and young would be vulnerable to predators. Another example of a commensal relationship is the clownfish and the sea anemone. The sea anemone is not harmed by the fish and the fish benefits from protection from predators who would be stung upon nearing the sea anemone.
Mutualism
The second type of symbiotic relationship is called mutualism, where two species benefit from their interaction. For example, termites have a mutualistic relationship with protozoa that live in the insect’s gut (a). The termite benefits from the ability of bacterial symbionts within the protozoa to digest cellulose. The termite itself cannot do this, and without the protozoa, it would not be able to obtain energy from its food (cellulose from the wood it chews and eats). The protozoa and the bacterial symbionts benefit by having a protective environment and a constant supply of food from the wood chewing actions of the termite. Lichens have a mutualistic relationship between fungus and photosynthetic algae or bacteria (b). As these symbionts grow together, the glucose produced by the algae provides nourishment for both organisms, whereas the physical structure of the lichen protects the algae from the elements and makes certain nutrients in the atmosphere more available to the algae.
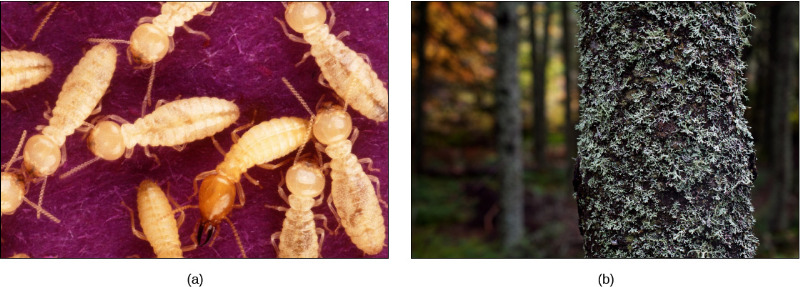
Parasitism
A parasite is an organism that lives in or on another living organism and derives nutrients from it. In this relationship, the parasite benefits, but the host is harmed. The host is usually weakened by the parasite as it siphons resources the host would normally use to maintain itself. The parasite, however, is unlikely to kill the host, especially not quickly, because this would allow no time for the organism to complete its reproductive cycle by spreading to another host.
The reproductive cycles of parasites are often very complex, sometimes requiring more than one host species. A tapeworm is a parasite that causes disease in humans when contaminated, undercooked meat is consumed. d\The tapeworm can live inside the intestine of the host for several years, benefiting from the food the host is eating and may grow to be over 50 ft long by adding segments. The parasite moves from species to species in a cycle, making two hosts necessary to complete its life cycle.
Another common parasite is Plasmodium falciparum, the protozoan cause of malaria, a significant disease in many parts of the world. Living in the human liver and red blood cells, the organism reproduces asexually in the gut of blood-feeding mosquitoes to complete its life cycle. Thus malaria is spread from human to human by mosquitoes, one of many arthropod-borne infectious diseases.
Review Question:
Cows have prokaryotic organisms (archaea) that live in their gut and aid in the digestion of grasses:_______
Summary
Communities include all the different species living in a given area. The variety of these species is called species richness. Many organisms have developed defenses against predation and herbivory, including mechanical defenses, warning coloration, and mimicry, as a result of evolution and the interaction with other members of the community. Two species cannot exist in the same habitat competing directly for the same resources. Species may form symbiotic relationships such as commensalism or mutualism.
End of Section Review Questions:
REVIEW: Competition
Thinking about it
Thinking about it
This is where you can add appendices or other back matter.
In biology, the fitness of an organism has to do with the number of offspring produced by their offspring (or the number of grandchildren an organism has). Therefore, the "fittest" organism has more grandchildren than the other organisms to which you are comparing it. It is true that increased survivorship correlates with increased fitness, but not always. An organism that lives for 100 years may only produce 5 grandchildren while an organism that lived for 20 years might produce 10 grandchildren. The shorter-lived individual would be considered "fittest" in this case. Therefore, survival is no guarantee of being the fittest.