2 What do ecologists study?
When you go outside, take note of the organisms around you. At first, they will seem to be all alike; every tree seems like every other, with a trunk, branches, and leaves all fed by an underground root system. After a closer examination, differences soon become apparent, until it seems as though there are no similarities between individuals at all; each squirrel is a different size and behaving differently from all of the others and even each leaf seems slightly different. When taken as a whole, however, patterns will emerge in the behaviors, morphology, and geography of the organisms in the world; different kinds of trees are found on hilltops and others in valleys; the squirrels in one location might be bigger than those in another. Some birds tend to stick to the interior of the forest, while others are found mostly along the edges. Some of this variation seems obvious and unsurprising, and other aspects are subtle and require detailed measurements to uncover.
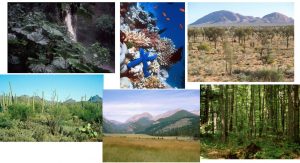
Living systems bear several major hallmarks, one of which is variation. While some variation is due to genetic differences among individuals, and some is due to differences in growth and development, there is a third cause of variation that must be taken into account. this is the external factors affecting individual organisms; in other words, the environment. Like many other aspects of biology, organisms and their environments form a complex set of interactions that feedback on each other.
2.1 The Theory of Ecology
The general theory of ecology consists of eight fundamental principles listed below. The roots of these principles can be traced to the origins of the science of ecology in the 19th century, the roots of which can be traced back through the study of natural history to ancient Greece. The word “ecology” comes from the Greek word “oîkos,” meaning “house,” indicating that ecology is the study of an organism’s abode.
The eight fundamental principles of the general theory of ecology
- Organisms are distributed unevenly in space and time.
- Organisms interact with their abiotic and biotic environments.
- The distributions of organisms and their interactions depend on contingencies (chance events).
- Variation in the characteristics of organisms results in variation of ecological patterns and processes.
- Environmental conditions, as perceived by organisms, are heterogeneous in space and time.
- Resources, as perceived by organisms, are finite and heterogeneous in space and time.
- Birth rates and death rates are a consequence of interactions with the abiotic and biotic environment.
- The ecological properties of species are the result of evolution.
We will explore all of these fundamental principles throughout this textbook. Each principle is addressed briefly and individually below, but throughout the rest of the book, you will see that these principles are tightly intertwined. These principles are presented first to give you a sense of how the ecological information you will learn in this book relates.
Ecology emerged as its own discipline between 1880 and 1910, along with much of biology. The roots of many of today’s ecological theories can be found in this period, although the fundamental principles emerged only slowly over the next 50 years. While all of the fundamental principles had been put forth by the middle of the 20th century, there were still serious debates about their relative importance. For example, the process of evolution in shaping global patterns of species distributions was recognized by Charles Darwin in the nineteenth century, but it was not until after the Modern Synthesis (see chapter 3) shaped the theory of evolution in the middle of the 20th century that there was extensive study of the interplay of ecological and evolutionary processes within local settings.
The domain of the science of ecology is the spatial and temporal patterns of the distribution and abundance of organisms, including causes and consequences. Strikingly, four of the eight fundamental principles are about variation of either the biotic or abiotic world. It is this focus on variation that makes ecological studies so challenging and exciting. In addition, ecology is the aspect of biology that pays the most attention to the world outside of the organism.
Because the science of ecology deals with interactions between organisms and the rest of the world, it is often linked with political concerns about the state of the world, which can be grouped under the heading of environmentalism. However, ecology is not environmental advocacy or political activism, although scientists are sometimes environmental activists in their personal lives, and environmental activists may, and should, rely on scientific research. Ecology itself is not about one’s feelings about nature, although ecologists may have strong feelings about what they study.
2.2 Principle 1: Uneven distributions
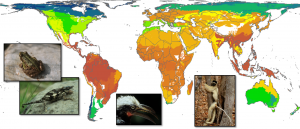
Organisms are not uniformly distributed across the face of the Earth. As far back as 2500 years ago, Greek natural historians were aware that different species were limited in the types of habitats in which they were found. However, scientists first became aware of large-scale patterns in species diversity as a result of the voyages of exploration and colonization undertaken by Europeans in the 18th and 19th centuries. Their ships often carried botanists and zoologists as part of the crew, the most famous example being the voyage of the Beagle (1831–1836), on which Charles Darwin served as the ship’s naturalist. These naturalists made records of the plants and animals they found, brought back specimens, and added them to the growing catalog of described species.
From these records, it soon became apparent that tropical regions were typically very rich in species, polar regions were very species-poor, and temperate regions had intermediate numbers of species. Brazil alone has over 56,000 named plant species, while the United States has about 18,000, and Canada has about 4200. An explanation for this pattern of more species in the tropics may be the oldest major ecological hypothesis. Between 1799 and 1804, Baron Alexander von Humboldt traveled through Mexico, Central America, and northwestern South America. He subsequently published a series of essays under the title Ansichten der Natur (“Views of Nature”), in which he described this pattern and postulated that it was due to differences in climate, specifically winter temperatures and the effects of freezing.
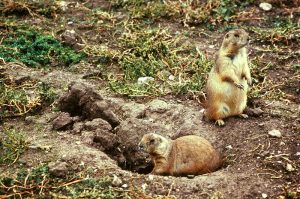
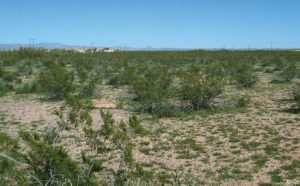
Even within a single population of one species, individuals generally are not uniformly distributed. They may be concentrated in patches, as with prairie dogs in the western United States. Prairie dogs live in large colonies called towns; in northern Colorado, each town contains about 850 individuals and the towns are separated by an average distance of 50 km. Individuals of a species may also be evenly spread throughout the landscape, as is seen with populations of creosote bushes in the Sonoran Desert that are spaced out because of competition for water. Thisvariation in abundance extends even to the smallest species. Within your body are thousands of species of bacteria, with different species living in different places. Some reside exclusively in your stomach and intestine, while others cruise your blood vessels. Just within your mouth, each tooth harbors its own set of bacterial species and those species remain for months no matter how much you brush your teeth.
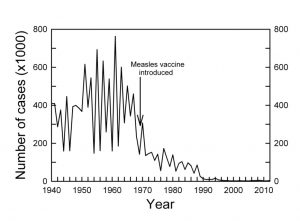
The number of individuals may also vary through time. For example, prior to the development of vaccines, many disease-causing viruses showed similar boom-and-bust cycles, with a sharp decline in the number of cases after vaccines were introduced. Not all change is cyclical, however. Following a major disturbance, such as a forest fire or a hurricane, the plants in a community will slowly change in composition. Immediately after the disturbance, some plants will resprout from still-living root systems and others will germinate from seeds that sat dormant in the soil or that are newly arrived at the site. The result can be a very different mix of species than were in that site prior to the disturbance. Over time the first species may be displaced by later arriving ones, until over the course of decades or centuries the original community reappears.
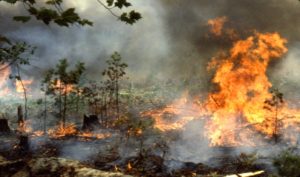
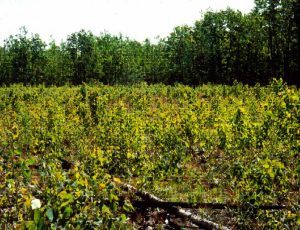
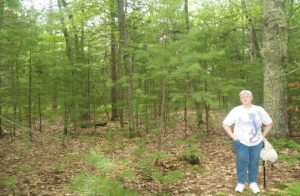
Change also occurs over much longer time spans. For the past 2 million years the Earth has been in the midst of a glacial period. During that time glaciers have waxed and waned at least four times. In North America, at the height of the last advance, glaciers extended as far south as the region around the Great Lakes. At that time forests were found only in what is now the southern United States. For the past 20,000 years, we have been in a period of glacial retreat and those forests have migrated to northern Canada. Over even longer time spans, however, some patterns are extremely stable. For example, the pattern of many species in the tropics and few at the poles is very old, going back at least to the time of the dinosaurs 100 million years ago.
Thus, the number of individuals in a population or the number of species in a community are dynamic. This dynamic aspect of ecological systems contrasts with an older view of the world existing in a “balance of nature,” an idea which goes back at least 2500 years to the Greek historian Herodotus, and was the prevailing view into the first half of the 20th century. While scientists recognized that communities changed following a disturbance, the notion was that communities tended to return to an equilibrium in which all plants and animals were at numbers sufficient to sustain the entire system. These ideas were most notably put forward in modern science by Fredric Clements, who formulated the first theory of change in plant communities. We now know that the idea that nature is in balance is wrong. Not only are systems dynamic, populations are often disappearing from a given site and replaced by other species or perhaps reappearing as new individuals move back into the system.
2.3 Principle 2: Biotic and abiotic interactions
All organisms live within a larger environment and, by necessity, interact with that environment; these interactions in turn determine the distribution and abundance of organisms. For simplicity, we can divide those interactions into ones involving the abiotic (non-living) parts of the environment, and those involving the biotic (living) parts. The nature of those interactions vary depending on whether the organism is a plant or an animal, single-celled or multicellular, and more, but the basic nature of those interactions is the same for all species.
One common aspect is how the environment affects an individual’s body temperature. For example, when you jump into water that is cooler than you are, you feel chilly. You do not get cold as quickly as a smaller animal would, however, because your greater mass stores more heat energy. If you were to begin swimming vigorously, the metabolic energy produced by your muscles from burning stored calories would warm you up. Because you are a mammal, you maintain a constant body temperature using that metabolic energy. Most organisms, however, do not. Their body temperature depends on absorbing energy from the environment, energy which we label “heat” or “light.” This is just one example of organisms’ interactions with the abiotic environment.
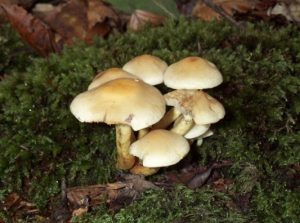
An example of a biotic interaction is when an organism consumes others for food usually by either consuming it while still alive, killing the other organism, or eating an organism that another animal has just killed or has recently died. This can include a group of lions chowing down on a zebra , or the vultures that feast on the carcass once the lions are done. Such consumption is called predation. Some organisms take in organic molecules from other organisms that are already dead, or from dead tissues such as the skin that you are constantly shedding. These latter organisms include most fungi and many bacteria.
When one organism eats another, the eater gets a benefit while the eaten suffers a cost. Many times that cost is death, but not always. When you harbor a cold virus, it will reproduce inside you and get passed on to others while only causing you a few days of illness. Most of the time when a plant gets eaten, only part of the plant gets taken, such as a caterpillar munching on a leaf. Thus, the amount of benefit and cost to each of the participants in the interaction can vary. Nor are interactions always of the type where one participant benefits while the other suffers a cost. Many types of interactions benefit both participants and are termed mutualisms. An obvious example is when a hummingbird visits a flower. The hummingbird drinks the nectar from the flower, a solution very high in sugar. At the same time, the hummingbird gets pollen deposited on its head. When the hummingbird visits the next flower, the pollen from the first gets left behind. Importantly, that next flower will generally be one of the same species, so that the pollen can fertilize that flower. Thus, the hummingbird gains energy and the flower gets its pollen moved to exactly the right place.
All of the interactions just described are direct, one individual doing something to another. But interactions can also be indirect. For example, there is a finite number of zebras, and if one gets eaten by a lion, that leaves fewer zebras for other lions or hyenas to eat. Indirect effects can also occur through changes in the abiotic environment. An especially dramatic example involves the actions of beavers. Beavers cut down trees and use the wood to build dams across streams, which causes the surrounding area to flood. Just like a manmade dam and attendant reservoir, this creates ponds within which the beavers build houses – creating a place protected from predators. In doing so, they change the environment. By flooding an area, they increase the amount of water in the soil around the pond as well as the amount of light on the area by removing the trees. Some plant species grow much better in areas with lots of light and very wet soil, so they indirectly benefit from the beavers’ activities, while other species are excluded by those changes. While ecologists have always worked to understand these interactions, such efforts were greatly enhanced with the movement towards descriptions through mathematical models. These interactions and their impacts on all levels of organization will be covered in more detail in later chapters.
2.4 Principle 3: Contingency
Chance can play both a small and large role in the abundance and distribution of organisms. Consider a field with many different
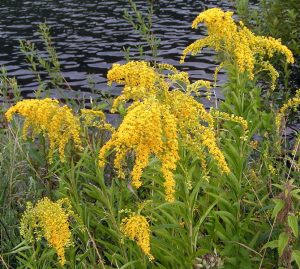
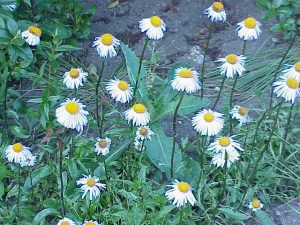
species of plants, including grasses and wildflowers, such as goldenrod. Each year the goldenrod individuals produce seeds that get blown about in the wind, landing in various places in the field. Some of those places are not near any other plants, and thus are good places to germinate and grow; other seeds will end up next to or directly underneath another plant, so the plants that germinate from those seeds may not grow very big or may simply die. Chance dictates how many seeds land in good places and which seeds those are. Once the seed starts to grow it must compete with the other plants around it for light and for the water and nutrients in the soil. Goldenrod is very different in form from the grasses in that field, and so does not compete very much with them. However, say that asters also grow in that field. Those plants are similar to goldenrods and so compete a bit more. And, of course, two goldenrods growing next to each will compete quite a bit. In addition to simple placement, which species an individual happens to be growing next to also depends a great deal on chance.
At much larger scales, the same sort of process can be seen. On the continent of Australia, the dominant mammals are marsupials like kangaroos and koalas. Placental mammals like humans and horses had not spread to Australia before that continent was separated from Africa, South America, and Antarctica. That chance event led to many differences in the animals of Australia and a wide-range of animals, such as koalas, kangaroos, wallabies, and Tasmanian devils, found only in Australia. Up until 40,000 years ago, the largest plant-eater was the Diprotodon. It grew to about 3 m in length and 2 m in height and weighed about 2500 kg. It mostly ate leaves of trees and shrubs and some grass. In many ways, it was similar to an elephant, but not as large.
Ecologists have debated for many decades about the importance of chance. For the most part, in the first half of the 20th century, most ecologists thought that chance played a relatively small role, although there were always some dissenters. The most vigorous debates were about the role of chance in changes in population numbers, a debate that was renewed about once a decade. This debate intensified during the 1960s and spread to other aspects of individual and species numbers and distributions; as it spread, this debate spurred the rapid development of mathematical models through which the effects of chance could be tested. Finally, by the 1980s most ecologists agreed that chance events could play a significant role in most ecological processes. It is no coincidence that this increase in the appreciation for the importance of chance happened at the same time that computers were becoming faster and easier to use. Models that incorporate random variation require fast computers for implementation. This linkage of computer technology and a change in ecological theory is an example of how changes in technology can drive scientific advancement.
2.5 Principle 4: Variation Among Individuals
No two individuals are ever identical. Even individuals that are genetically identical grow up under slightly different circumstances resulting in differences in form or behavior. Differences become more and more apparent the more genetic variation is involved: collections of individuals, populations, will also differ from each other, and most noticeable are the differences between species, many of which are caused by natural selection (see Chapter 3). All of these types of variation can affect ecological patterns and processes and understanding those effects can have important practical implications when it comes to species conservation.
Because population numbers naturally cycle up and down, one cause of local extinction of that population is if the changes in the environment lead to the death of just a few more individuals during one of those down phases, causing the population numbers to drop to zero. For example, an especially cold winter may result in a few more individuals freezing to death, or a bad drought may mean more plants die from insufficient water. If all of the individuals in a population were identical, then the chance of every individual dying in especially adverse conditions would increase. However, if during that drought, some of the plants are more resistant to drying out or better able to tolerate drying out, then their chance of survival will be greater and at least some individuals will survive. Then, when conditions improve the population numbers will again rise.
Variation among species can have similar effects on the stability of entire communities. For example, plant species differ in their ability to grow given different amounts of water. Some species grow best under drier conditions, while others grow best under wetter conditions. Since rainfall can vary substantially from one year to the next, a plant community that contains a diverse array of species varies less in total growth from year to year than a community consisting of just one or two species. In the diverse community, at least a few species can grow well under any given amount of rainfall, thus maintaining a minimum biomass. Species diversity alone, however, is not the only factor that affects the structure of communities. As food webs increase in number of species, they can become more sensitive to small disturbances because a change in the number of individuals in one species quickly affects all of the other species. However, if the food web consists of some species that are tightly linked to each other (e.g., a predator that specializes on a single prey species) and others that are only loosely linked (e.g., a predator that eats lots of different species so that it does not rely on any single prey species), then changes in species numbers in one part of the food web do not affect those in other parts.
2.6 Principle 5: Environmental conditions
Variation in a species’ location is caused by many factors, one of which is variation in the environment. As shown above, the greatest numbers of species are found in equatorial regions and the fewest numbers are found in polar regions and deserts. This variation in species numbers is associated with differences in annual temperatures and rainfall. In general, more species are found in warmer and wetter environments. This pattern of differences in temperature and rainfall corresponding to differences in species are also found across continents and even at distances of tens of kilometers. In addition to distance, time also creates variations; the environment varies in the same site from day to night, from week to week, and across seasons. The Earth itself has been subject to much longer cycles, such as the advance and retreat of glaciers over tens of thousands of years.
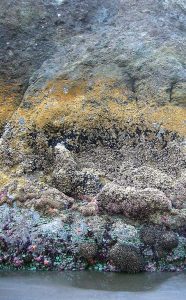
All aspects of the environment vary, each having its own effects on the distribution of organisms. At the ocean’s shore, for example, are places that are always underwater, others that are dry part of the day and wet at other times as the tides go up and down, and others that only occasionally get wet, leading to very different species in those various zones. Few plants can grow in soils with high amounts of salt, so the spray from the waves limits which species grow near the shore.
Environmental variation occurs at very short distances and across the entire globe, from moment to moment and from aeon to aeon. As dramatic as this variation can be, not every aspect of it affects all species equally. The relative importance depends on the organism; what a bacterium perceives is not what an elephant perceives. For a bacterium, differences in soil nutrients over distances of micrometers can be critical, a distance that would make no difference to an elephant. A squirrel may live and die within a year, while the oak tree that it climbs might live for hundreds of years. Changes in climate that take decades to happen are not experienced by the squirrel, but can be vital to the life of the oak tree.
These large differences in the scale and pace of environmental variation pose challenges to scientists trying to understand the interaction between organisms and their environment. For small organisms, experiments can be done in a laboratory or greenhouse. For global-scale processes, manipulative experiments are not possible, so scientists must rely on mathematical models using correlations between environmental factors and species distributions to formulate these larger-scale theories. Such observational experiments have limitations in what they can tell us about causal mechanisms and are best when they can be paired with manipulative experiments. In the past few decades, scientists have been performing much larger and longer manipulative experiments that have provided new insights into ecological processes.
2.7 Principle 6: Finite resources
An important distinction among types of environmental factors are conditions versus resources. Conditions are unaffected by organisms – for example, temperature is a condition in that it affects the organism, but no matter how warm or cold the organism gets the organism does not increase or decrease the amount of heat in the broader surrounding environment. In contrast resources are finite and potentially depleted by organisms. If foxes are eating rabbits, there are only so many rabbits to go around. Every rabbit eaten means one fewer available for another animal to eat. Resources, therefore, are subject to competition, unlike environmental conditions; both environmental conditions and resources, however, vary in space and time. The heterogeneity of resources has a number of causes. Variation in environmental conditions can have indirect effects on the distribution and abundance of species through their affects on organisms that serve as resources. The processes that cause variation in resources are often the same as those that cause variation in environmental conditions. For example, seasonal variation in light and temperature are caused by the movement of the Earth around the Sun. However, light is subject to competition (e.g., when one plant shades another) whereas temperature is a condition and not subject to competition.
Whether a particular environmental factor is a condition or a resource is context dependent. For example, water is sometimes a resource subject to competition (e.g., plants in a desert) and sometimes a condition (e.g., fish in the ocean). Some elements (e.g., manganese) can be limiting to plants at low levels, thereby acting as a resource. On the other hand, that same element can be toxic at high levels, in that case acting as a condition. Thus, while the distinction between conditions and resources is important, the two categories blur into each other. Both resources and conditions control where a species can live, but only resources are capable of being depleted.
2.8 Principle 7: Birth and death
The evolutionary fitness of an organism is the result of that individual’s life history and reproduction (see Chapter 3). An organism’s birth and death processes and rates are at least partially dependent on the environment of the organism, both conditions and resources.
For example, reproduction requires resources to build new cells beyond those needed for survival. The rate at which an organism reproduces – for example, how quickly a single-celled organism can divide or how many eggs a bird can lay – depends on the rate at which that individual can take up nutrients from the environment. It is more than simply how fast an animal can eat, however. Often, one particular nutrient, such as phosphorus, will be the limiting factor. Sometimes the limiting resource is other individuals of the same species. The limitation may be due to competition or, in some circumstances where individuals are widely separated, such as tigers where each adult male or female will control a large territory, simply finding another individual to mate with can create limitations on the rate of reproduction. Death rates similarly also depend on availability of resources, environmental conditions and the presence of other species.
Competition for limited resources determines the number of individuals that can live in an area. Such competition can occur between individuals within a species – Canada lynx competing for snowshoe hares – or among different species – arctic foxes eating those same hares. If the persistence of a population depends on it being above some minimum size, the amount of available food would limit not just the number of individuals but also the number of species. Consider, for example, if the minimum population size of both the lynx and fox was 100 individuals; there would have to be enough hares for 200 individuals for both species to be able to persist in an area. If there were only enough hares for 120 individuals, then one species would go locally extinct.
2.9 Principle 8: Evolution
The process of change over generations (evolution) and environmental interactions (ecology) are intertwined. The biology of organisms creates the context within which evolution occurs and, in turn, evolution determines the properties of organisms. The environment creates context in two ways. First, the characteristics of an organism are determined both by the environment within which the organism’s genes are expressed and the organism’s genes. In other words, the appearance and other characteristics of an organism are determined both by the organism’s genetics and its environment. Second, the relationship between the characteristics of an organism and its fitness is determined by the organism’s abiotic and biotic environment; thus, the direction of natural selection is a function of the environment (see Chapter 3).
It may seem obvious that the ecology of an organism has been shaped, at least in part, by its evolutionary history. But such evolutionary thinking was not always common among ecological scientists. The inclusion of evolution within ecological thinking was an important outcome of the Modern Synthesis (see Chapter 3). Although evolutionary thinking about ecological processes goes back at least to Darwin, evolutionary thinking began infusing ecology in a significant way in the 1920s and its widespread acceptance occurred primarily in the latter half of the 20th century.
Even today, most ecological studies do not take into account evolutionary processes. In some instances, evolution can be safely ignored, particularly when the time period consider includes only a few generations of the studied species. On the other hand, when studying very long time periods or species with short generation times (the time between successive generations), evolutionary processes may be important. For example, cycles of measles outbreaks may be affected by evolution, as even within a single person the measles virus is going through many generations allowing many opportunities for mutation and new genetic variation.
Such interactions of ecology and evolution can be seen when two species interact and evolve in response to each other. Consider the case of the Australian wild flax plant and a fungus that can infect it, causing flax rust. A long-term study was undertaken in southeastern Australia beginning in 1986. In 1990, the population being monitored suddenly crashed, dropping from a density of 22.6 individuals/m2 to 8.5 individuals/m2 in just one year. A new strain of rust had appeared in the population, one to which most plants were susceptible. However, within a single year the percentage of the population that was resistant to that new strain increased from about 3% to over 20%, and as a result the numbers of individuals in the population began to climb[1]. This change in the average resistance of the population is an example of very fast evolution in response to strong natural selection.
These principles will be explored in more depth through the textbook, beginning with an exploration of evolution.
Levels of Ecological Study
Within the discipline of ecology, researchers work at four general levels, which sometimes overlap. These levels are organism, population, community, and ecosystem
Organismal Ecology
Researchers studying ecology at the organismal level are interested in the adaptations that enable individuals to live in specific habitats. These adaptations can be morphological, physiological, and behavioral. For instance, the Karner blue butterfly (Lycaeides melissa samuelis) (Figure 44.3) is considered a specialist because the females only oviposit (that is, lay eggs) on wild lupine (Lupinus perennis). This specific requirement and adaptation means that the Karner blue butterfly is completely dependent on the presence of wild lupine plants for its survival.
After hatching, the (first instar) caterpillars emerge and spend four to six weeks feeding solely on wild lupine (Figure 44.4). The caterpillars pupate as a chrysalis to undergo the final stage of metamorphosis and emerge as butterflies after about four weeks. The adult butterflies feed on the nectar of flowers of wild lupine and other plant species, such as milkweeds. Generally there are two broods of the Karner blue each year.
A researcher interested in studying Karner blue butterflies at the organismal level might, in addition to asking questions about egg laying requirements, ask questions about the butterflies’ preferred thoracic flight temperature (a physiological question), or the behavior of the caterpillars when they are at different larval stages (a behavioral question).
Population Ecology
A population is a group of interbreeding organisms that are members of the same species living in the same area at the same time. (Organisms that are all members of the same species are called conspecifics.) A population is identified, in part, by where it lives, and its area of population may have natural or artificial boundaries. Natural boundaries might be rivers, mountains, or deserts, while artificial boundaries may be mowed grass, manmade structures, or roads. The study of population ecology focuses on the number of individuals in an area and how and why population size changes over time.
For example, population ecologists are particularly interested in counting the Karner blue butterfly because it is classified as a federally endangered species. However, the distribution and density of this species is highly influenced by the distribution and abundance of wild lupine, and the biophysical environment around it. Researchers might ask questions about the factors leading to the decline of wild lupine and how these affect Karner blue butterflies. For example, ecologists know that wild lupine thrives in open areas where trees and shrubs are largely absent. In natural settings, intermittent wildfires regularly remove trees and shrubs, helping to maintain the open areas that wild lupine requires. Mathematical models can be used to understand how wildfire suppression by humans has led to the decline of this important plant for the Karner blue butterfly.
Community Ecology
A biological community consists of the different species within an area, typically a three-dimensional space, and the interactions within and among these species. Community ecologists are interested in the processes driving these interactions and their consequences. Questions about conspecific interactions often focus on competition among members of the same species for a limited resource. Ecologists also study interactions between various species; members of different species are called heterospecifics. Examples of heterospecific interactions include predation, parasitism, herbivory, competition, and pollination. These interactions can have regulating effects on population sizes and can impact ecological and evolutionary processes affecting diversity.
For example, Karner blue butterfly larvae form mutualistic relationships with ants (especially Formica spp). Mutualism is a form of long-term relationship that has coevolved between two species and from which each species benefits. For mutualism to exist between individual organisms, each species must receive some benefit from the other as a consequence of the relationship. Researchers have shown that there is an increase in survival when ants protect Karner blue butterfly larvae (caterpillars) from predaceous insects and spiders, an act known as “tending.” This might be because the larvae spend less time in each life stage when tended by ants, which provides an advantage for the larvae. Meanwhile, to attract the ants, the Karner blue butterfly larvae secrete ant-like pheromones and a carbohydrate-rich substance that is an important energy source for the ants. Both the Karner blue larvae and the ants benefit from their interaction, although the species of attendant ants may be partially opportunistic and vary over the range of the butterfly.
Ecosystem Ecology
Ecosystem ecology is an extension of organismal, population, and community ecology. The ecosystem is composed of all the biotic components (living things) in an area along with the abiotic components (nonliving things) of that area. Some of the abiotic components include air, water, and soil. Ecosystem biologists ask questions about how nutrients and energy are stored and how they move among organisms and through the surrounding atmosphere, soil, and water.
The Karner blue butterflies and the wild lupine live in an oak-pine barren habitat. This habitat is characterized by natural disturbance and nutrient-poor soils that are low in nitrogen. The availability of nutrients is an important factor in the distribution of the plants that live in this habitat. Researchers interested in ecosystem ecology could ask questions about the importance of limited resources and the movement of resources, such as nutrients, though the biotic and abiotic portions of the ecosystem.
Most content adapted with permission from:
Foundations of Biology (http://www.foundationsofbiology.com/)
- Samuel M. Scheiner and Kayla I. Scheiner
Copyright (c) 2013 by S. M. Scheiner and K. I. Scheiner. This work is made available under the terms of the Creative Commons Attribution-NonCommercial-NoDerivs 3.0 license (http://creativecommons.org/licenses/by-nc-nd/3.0/)
Levels of Ecological Study – adapted from OpenStaxs Biology 2e by Mary Ann Clark, Matthew Douglas, Jung Choi.
-
Access for free at https://openstax.org/books/biology-2e/pages/1-introduction
- Burdon, J., & Thompson, J. (1995). Changed Patterns of Resistance in a Population of Linum Marginale Attacked by the Rust Pathogen Melampsora Lini. Journal of Ecology, 83(2), 199-206. doi:10.2307/2261558 ↵