12 Chapter 12 The Nervous System and Nervous Tissue
By Aylin Marz
Motivation.
Drug addiction is a health issue that devastates individuals as well as whole communities including some African American communities. Risk factors that increase someone’s chance of becoming an addict are not fully understood but these include environmental and genetics factors that affect the nervous system. Addiction is a disease of the nervous system. Being addicted to a drug like cocaine changes the chemistry of the brain down to the level of the neurotransmitter chemicals that send signals between neurons. Dopamine is the feel good neurotransmitter that activates the pleasure centers of the brain in everyone but the amounts of it become too high in addicts. Understanding how the nervous system works to transmit signals and control these signals can help understand diseases like addiction and why they are so difficult to treat. As a health professional dealing with athletes who may have gotten hooked on opioids prescribed to them after surgery or trying to deal with whole communities devastated by addiction as a nurse in your community, it is crucial to know the biology and chemistry of the nervous system.
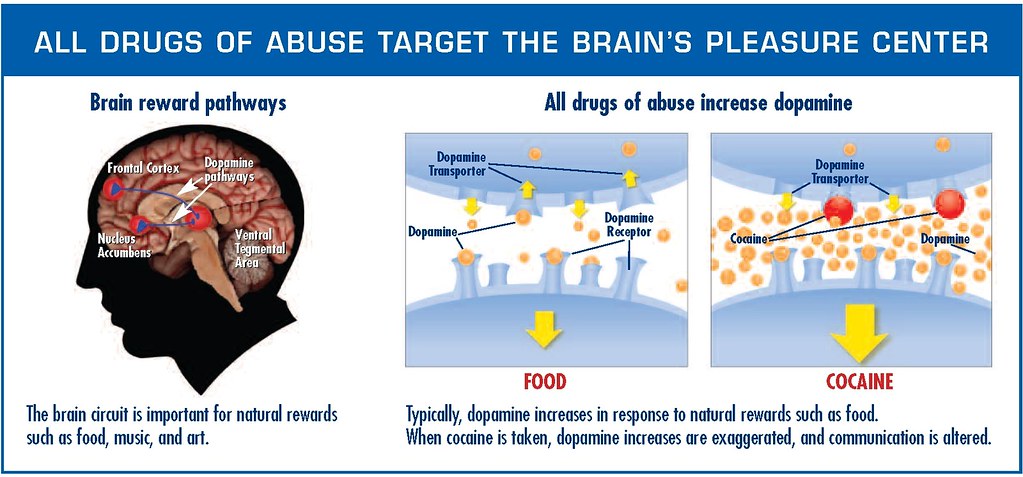
Learning Objectives
Upon completion of the work in this chapter students should be able to:
- Create a 3D model of a typical multipolar neuron to label structures such as dendrites, cell body, axon, myelin sheath and organelles such as the nucleus and mitochondria
- Perform microscopy to identify the gray and white matter areas and the neurons and glial cells within these areas using a spinal cord section
- Demonstrate an understanding of how action potentials are generated by interpreting the effects of mutant or blocked voltage-gated Na+ or K+ channels on action potentials
- Explain how a neurotransmitter works by identifying what goes wrong with the neurotransmitter dopamine in addiction
Background.
Basic Structure and Function of the Nervous System
The nervous system can be separated into divisions on the basis of anatomy and physiology. The anatomical divisions are the central and peripheral nervous systems (aka CNS and PNS, respectively). The CNS is the brain and spinal cord. The PNS is everything else. Functionally, the nervous system can be divided into those regions that are responsible for sensation, those that are responsible for integration, and those that are responsible for generating responses. All of these functional areas are found in both the central and peripheral anatomy.
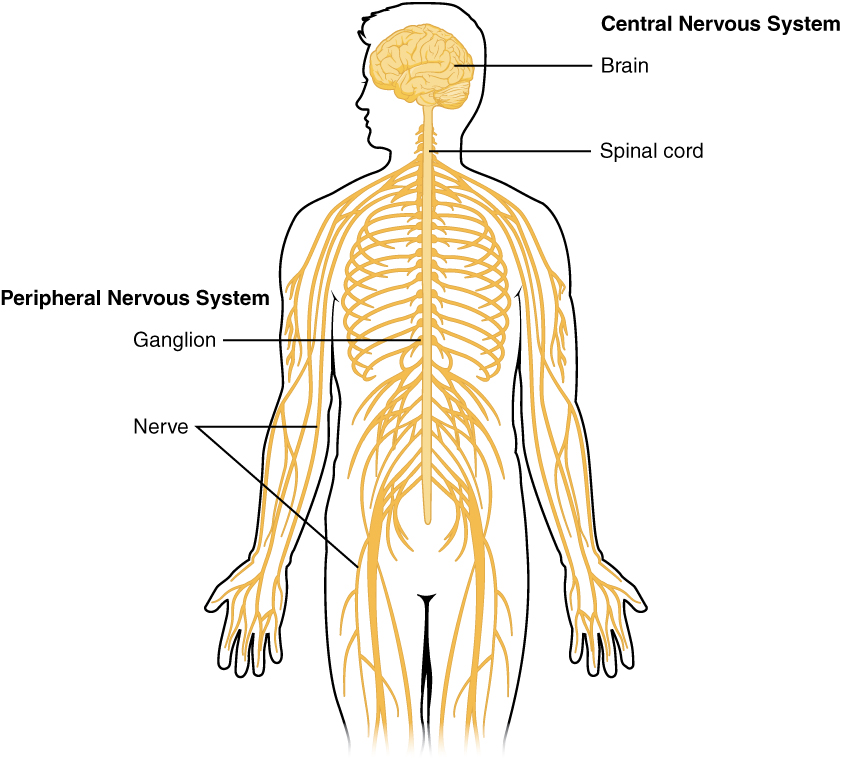
Considering the anatomical regions of the nervous system, there are specific names for the structures within each division. A localized collection of neuron cell bodies is referred to as a nucleus in the CNS and as a ganglion in the PNS. A bundle of axons is referred to as a tract in the CNS and as a nerve in the PNS. Whereas nuclei and ganglia are specifically in the central or peripheral divisions, axons can cross the boundary between the two. A single axon can be part of a nerve and a tract. The name for that specific structure depends on its location.
Nervous tissue can also be described as gray matter and white matter on the basis of its appearance in unstained tissue. These descriptions are more often used in the CNS. Gray matter is where nuclei are found and white matter is where tracts are found. In the PNS, ganglia are basically gray matter and nerves are white matter.
The nervous system can also be divided on the basis of how it controls the body. The somatic nervous system (SNS) is responsible for functions that result in moving skeletal muscles. Any sensory or integrative functions that result in the movement of skeletal muscle would be considered somatic. The autonomic nervous system (ANS) is responsible for functions that affect cardiac or smooth muscle tissue, or that cause glands to produce their secretions. Autonomic functions are distributed between central and peripheral regions of the nervous system. The sensations that lead to autonomic functions can be the same sensations that are part of initiating somatic responses. Somatic and autonomic integrative functions may overlap as well.
A special division of the nervous system is the enteric nervous system, which is responsible for controlling the digestive organs. Parts of the autonomic nervous system overlap with the enteric nervous system. The enteric nervous system is exclusively found in the periphery because it is the nervous tissue in the organs of the digestive system.
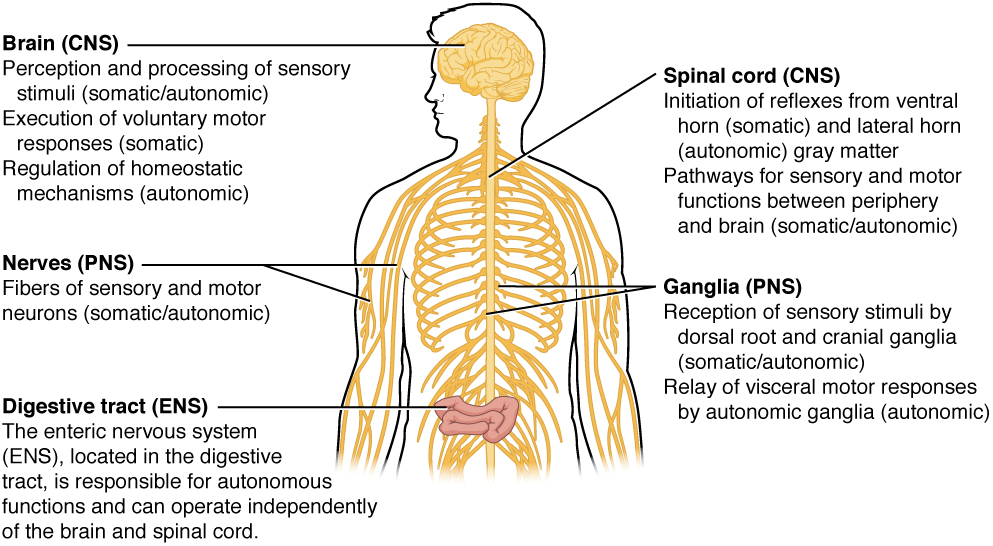
Nervous Tissue
Nervous tissue contains two major cell types, neurons and glial cells. Neurons are the cells responsible for communication through electrical signals. Glial cells are supporting cells, maintaining the environment around the neurons.
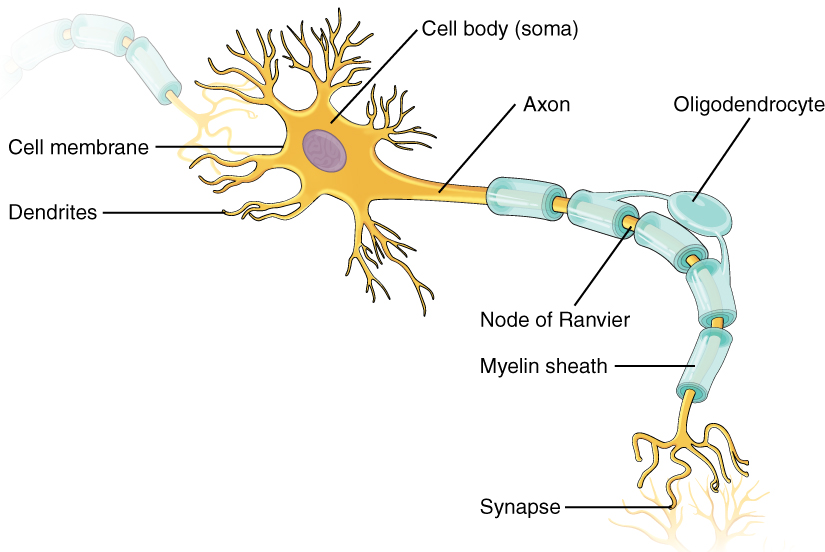
Neurons are polarized cells, based on the flow of electrical signals along their membrane. Signals are received at the dendrites, are passed along the cell body, and propagate along the axon towards the target, which may be another neuron, muscle tissue, or a gland. Many axons are insulated by a lipid-rich substance called myelin. Specific types of glial cells provide this insulation.
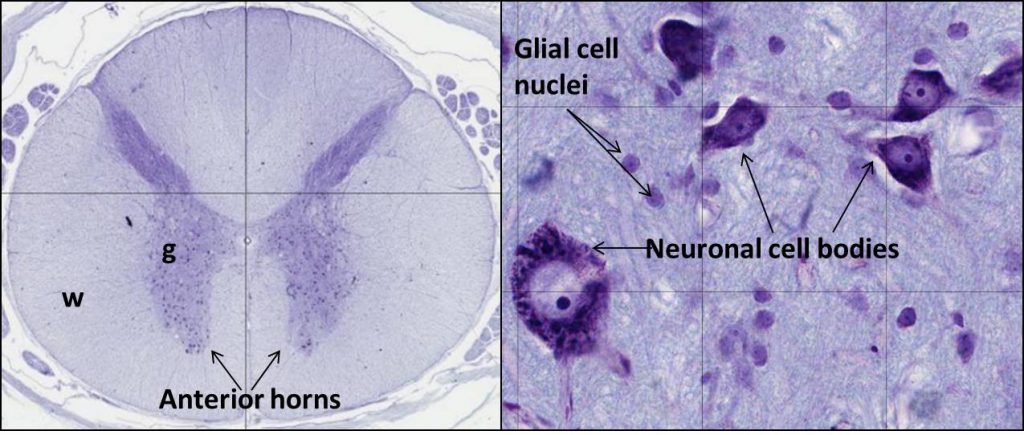
Several types of glial cells are found in the nervous system, and they can be categorized by the anatomical division in which they are found. In the CNS, astrocytes, oligodendrocytes, microglia, and ependymal cells are found. Astrocytes are important for maintaining the chemical environment around the neuron and are crucial for regulating the blood-brain barrier. Oligodendrocytes are the myelinating glia in the CNS. Microglia act as phagocytes and play a role in immune surveillance. Ependymal cells are responsible for filtering the blood to produce cerebrospinal fluid, which is a circulatory fluid that performs some of the functions of blood in the brain and spinal cord because of the blood brain barrier or BBB. In the PNS, satellite cells are supporting cells for the neurons, and Schwann cells produce myelin to insulate peripheral axons.
The Function of Nervous Tissue
Sensation starts with the activation of a sensory ending, such as the thermoreceptor in the skin sensing the temperature of the water. The sensory endings in the skin initiate an electrical signal that travels along the sensory axon within a nerve into the spinal cord, where it synapses with a neuron in the gray matter of the spinal cord. The temperature information represented in that electrical signal is passed to the next neuron by a chemical signal that diffuses across the small gap of the synapse and initiates a new electrical signal in the target cell. That signal travels through the sensory pathway to the brain, passing through the thalamus, where conscious perception of the water temperature is made possible by the cerebral cortex. Following integration of that information with other cognitive processes and sensory information, the brain sends a command back down to the spinal cord to initiate a motor response by controlling a skeletal muscle. The motor pathway is composed of two cells, the upper motor neuron and the lower motor neuron. The upper motor neuron has its cell body in the cerebral cortex and synapses on a cell in the gray matter of the spinal cord. The lower motor neuron is that cell in the gray matter of the spinal cord and its axon extends into the periphery where it synapses with a skeletal muscle in a neuromuscular junction.
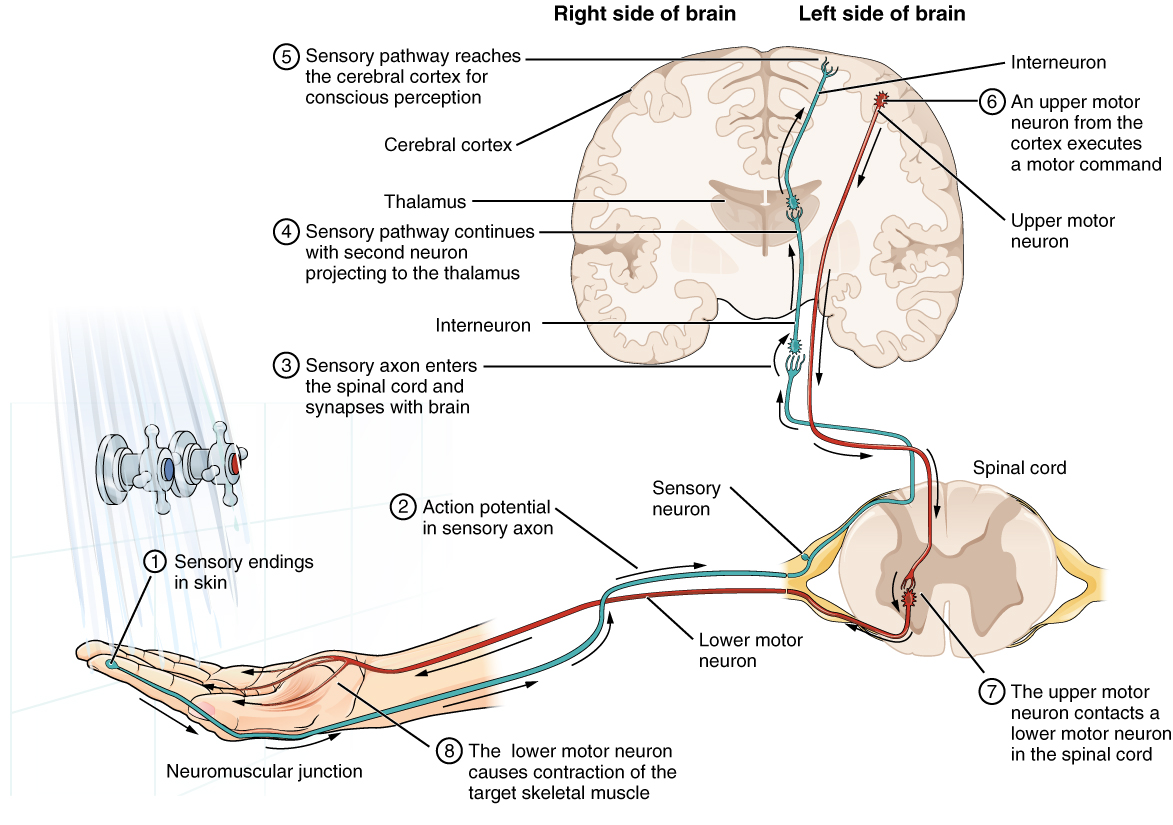
The Action Potential
The nervous system is characterized by electrical signals that are sent from one area to another. Whether those areas are close or very far apart, the signal must travel along an axon. The basis of the electrical signal is the controlled distribution of ions across the membrane. Transmembrane ion channels regulate when ions can move in or out of the cell, so that a precise signal is generated. This signal is the action potential which has a very characteristic shape based on voltage changes across the membrane in a given time period.
The membrane is normally at rest with established Na+ and K+ concentrations on either side. A stimulus will start the depolarization of the membrane, and voltage-gated channels will result in further depolarization followed by repolarization of the membrane. A slight overshoot of hyperpolarization marks the end of the action potential. While an action potential is in progress, another cannot be generated under the same conditions. While the voltage-gated Na+ channel is inactivated, absolutely no action potentials can be generated. Once that channel has returned to its resting state, a new action potential is possible, but it must be started by a relatively stronger stimulus to overcome the K+ leaving the cell.
The action potential travels down the axon as voltage-gated ion channels are opened by the spreading depolarization. In unmyelinated axons, this happens in a continuous fashion because there are voltage-gated channels throughout the membrane. In myelinated axons, propagation is described as saltatory because voltage-gated channels are only found at the nodes of Ranvier and the electrical events seem to “jump” from one node to the next. Saltatory conduction is faster than continuous conduction, meaning that myelinated axons propagate their signals faster. The diameter of the axon also makes a difference as ions diffusing within the cell have less resistance in a wider space.
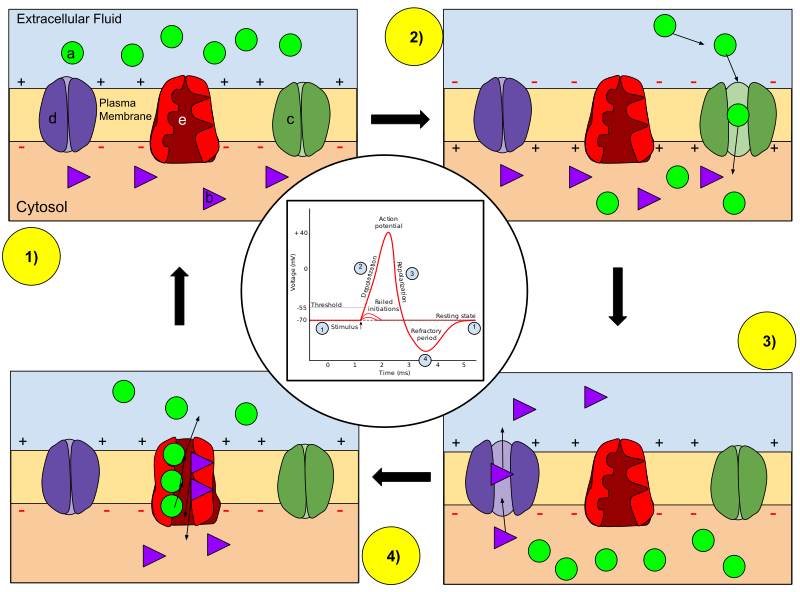
In the stages of an action potential, the permeability of the membrane of the neuron changes. At the resting state (1), sodium and potassium ions are unable to pass through the membrane, and the neuron has a negative charge inside (mainly due to the large proteins that are negatively charged, as well as the lower amount of positive K+ ions inside the neuron). Once the action potential is triggered, the depolarization (2) of the neuron activates the sodium channel, allowing sodium ions to pass through the membrane of the neuron and results in a positive charge in the neuron and a negative charge in the extracellular fluid. After the action potential is reached, the neuron begins repolarization (3), where the sodium channels close and the potassium channels open, allowing potassium ions to cross the membrane and flood into the extracellular fluid, resulting in a positive charge in the extracellular fluid and a negative charge that is below the resting potential of the neuron. Finally, the membrane potential returns to the resting state as the potassium channels close during the refractory period (4). The sodium-potassium pump works to maintain the concentration gradient over time by exchanging three sodium ions per two potassium ions across the plasma membrane.
View the Action Potential Animation for animated information.
Communication between Neurons
The basis of the electrical signal within a neuron is the action potential that propagates down the axon. For a neuron to generate an action potential, it needs to receive input from another source, either another neuron or a sensory stimulus. That input will result in opening ion channels in the neuron, resulting in a graded potential based on the strength of the stimulus. Graded potentials can be depolarizing or hyperpolarizing and can summate to affect the probability of the neuron reaching threshold.
Graded potentials can be the result of sensory stimuli. If the sensory stimulus is received by the dendrites of a sensory neuron, such as the sensory neuron ending in the skin, the graded potential is called a generator potential because it can directly generate the action potential in the initial segment of the axon. If the sensory stimulus is received by a specialized sensory receptor cell, the graded potential is called a receptor potential. Graded potentials produced by interactions between neurons at synapses are called postsynaptic potentials (PSPs). A depolarizing graded potential at a synapse is called an excitatory PSP (EPSP), and a hyperpolarizing graded potential at a synapse is called an inhibitory PSP (IPSP). Summation of EPSPs and IPSPs determine if the membrane reaches the threshold depolarization level of -55 mV from the resting potential of -70 mV.
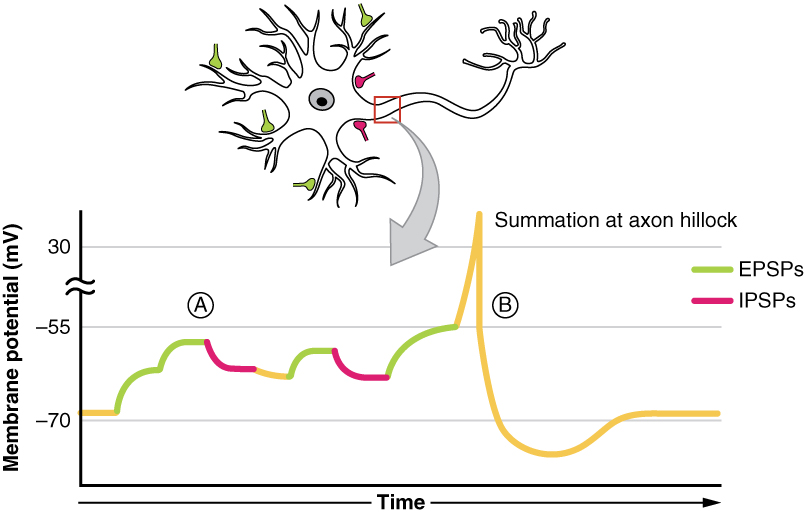
Synapses are the contacts between neurons, which can either be chemical or electrical in nature. Chemical synapses are far more common. At a chemical synapse, neurotransmitter is released from the presynaptic element and diffuses across the synaptic cleft. The neurotransmitter binds to a receptor protein and causes a change in the postsynaptic membrane (the PSP). The neurotransmitter must be inactivated or removed from the synaptic cleft so that the stimulus is limited in time.
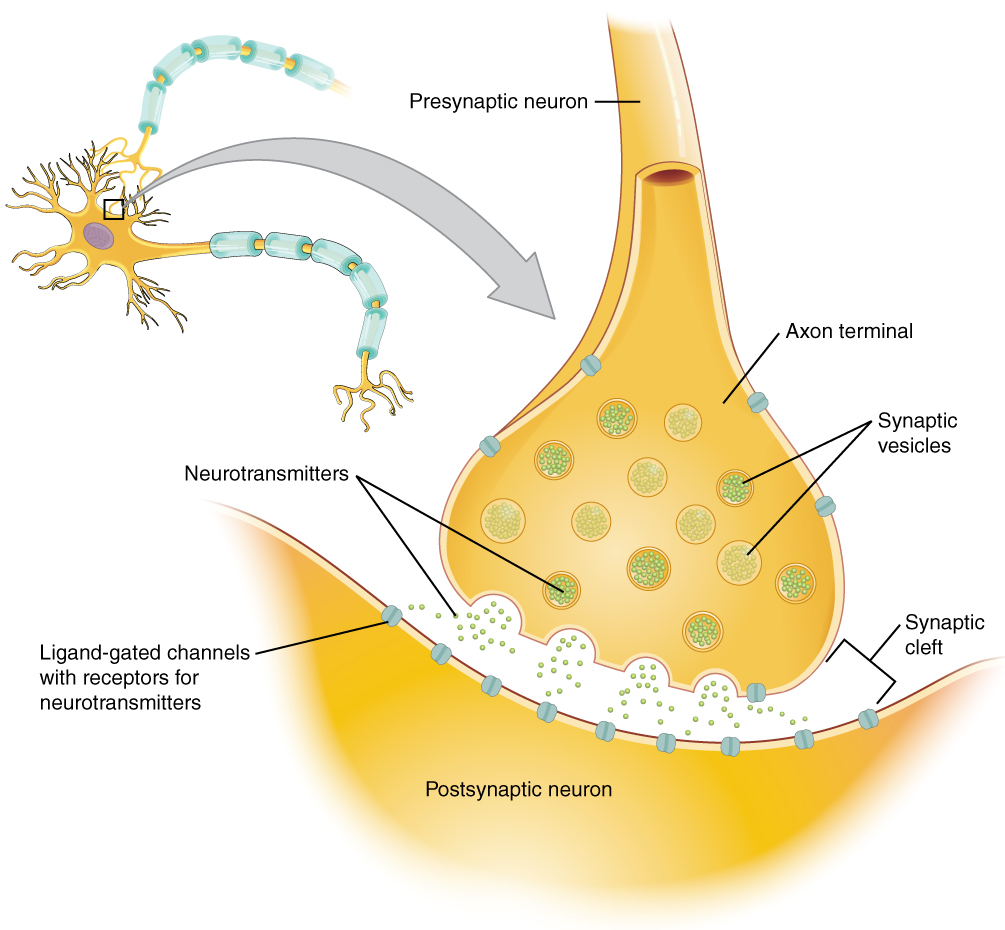
The particular characteristics of a synapse vary based on the neurotransmitter system produced by that neuron. The cholinergic system which uses acetylcholine (ACh) as a neurotransmitter is found at the neuromuscular junction and in certain places within the nervous system. Amino acids, such as glutamate, glycine, and gamma-aminobutyric acid (GABA) are used as neurotransmitters. Other neurotransmitters are the result of amino acids being enzymatically changed, as in the biogenic amines such as dopamine (reward neurotransmitter), or being covalently bonded together, as in the neuropeptides.
Pre-Laboratory Questions
After you review the Background information above, answer the following pre-laboratory questions before starting your lab exercises:
- What are the two anatomical divisions of the nervous system?
- List the three main functions of nervous system tissues.
- What do we call main functional cell types that transmit electrical signals in the nervous system?
- How is an action potential generated?
- Explain how signal is transmitted from a presynaptic to a postsynaptic neuron at the synapse.
Exercises
- Exercise 1 Create a 3D model of a multipolar neuron and label its structures and organelles
- Exercise 2 Sketch and identify neural tissue components by spinal cord section microscopy
- Exercise 3 Interpret effects on action potential of changes to voltage-gated Na+ channels
- Exercise 4 Predict the effect of manipulating the neurotransmitter dopamine on addiction
Exercise 1 Create a 3D model of a multipolar neuron and label its structures and organelles
Required Materials
- Plaster (multiple colors)
- Wired threads (multiple colors)
- Styrofoam balls
- Straws
- Wooden sticks
- Water color paint
- Plates
- Colorful Markers
- Construction paper
- Labeling Tape
- Labeling Pins
- Scissors
- Neuron models
Procedure
- Your task is to generate as complete a 3D model as possible. You may need to make more than one model to demonstrate both the gross structures and the smaller organelle type structures and detail within the neuron. When you are finished, you should have labelled the following structures on your 3D model.
- As you build your model, make sure to use the pins and labeling tape to carefully label as many of the above structures as possible. Have fun! When finished, present your model to your instructor. Leave the model or models on the instructor’s table or side bench for further examination.
- Take a picture of the labeled models and paste them below ( and in the Post-laboratory Questions section).
Gross structures:
- Dendrites
- Cell body
- Axon hillock
- Axon
- Myelin
- Nodes of Ranvier
- Synaptic knobs
Internal detail:
- Nucleus
- Nucleolus
- Cell membrane
- Cytoplasm
- Mictochondria
- Endoplasmic reticulum
- Golgi apparatus
- Cytoskeleton
- Axoplasm
- Axon cytoskeleton
- Synaptic vesicles with neurotransmitter
- Voltage-gated Na+ channels
- Voltage-gated K+ channels
- Voltage-gated Ca+ channels
- Ligand-gated Na+ channels
- Ligand-gated Cl- channels
Exercise 2 Sketch and identify neural tissue components by spinal cord section microscopy
Required Materials
- A slide of the cross section of the spinal cord
- A light microscope with 4x, 10x, 40x, and 100x objectives and 10x ocular objective
- Lens cleaning fluid
- Lens paper
- Microscope immersion oil
Procedure
- Nervous system tissues contain neurons that transmit electrical signals and glial cells that have supportive functions. In this exercise, you will examine the microscopic structure of the spinal cord which is part of the CNS (Figure 12.5). This will enable you to identify white and gray matter areas at low magnification, as well as neurons, myelinated axons, and glial cells at high magnification.
- Observe the spinal cord section using the lowest objective magnification (4x). Identify the white matter and gray matter areas of the spinal cord. Also identify the ventral (anterior) and dorsal (posterior) horns that are part of the gray matter.
- Observe neurons and glial cells at high magnification (10x, then 40x, and possibly with oil at 100x). Before you change your objective from 4x, first determine whether you should be looking in the gray matter or white matter areas. Then, move your slide to the correct matter area. Scan that area at higher magnification to find neuronal cell bodies and glial cell nuclei.
- Observe cross sections of myelinated axons at high magnification (10x, then 40x, and possibly with oil at 100x). First decide if you need to be examining the gray matter or white matter areas of the spinal cord section. Then, move into the appropriate area. Scan your slide to see if you can recognize a myelinated axon cross section which looks like a white halo around a dark circle.
- Sketch the low and high magnification images of the spinal cord section below and label all of these structures on them using the space below.
Exercise 3 Interpret effects on action potential of changes to voltage-gated Na+ channels
Required Materials
- Scenario: Lidocaine is a local anesthetic or numbing agent which prevents sensory neurons from sending pain signals to the brain. Lidocaine achieves its numbing effect by binding to and reversibly blocking voltage gated sodium Na+ channels. What “reversible” blocking means is that once the Lidocaine levels are reduced, the sodium Na+ channel function gets back to normal. Tetrodotoxin is another sodium Na+ channel blocker. However, tetrodotoxin is considered to be a neurotoxin. It is a naturally occurring toxin found in pufferfish, porcupine fish, ocean sunfish, and triggerfish. Unlike lidocaine, when tetrodotoxin blocks the sodium channel, this is not reversible. In this exercise, we will predict how these chemicals affect the initiation and progression of the action potentials down a neuron.
- Model of action potential: We will use the figure of action potential generation displayed in Figure 12.7 as a model
Key: (a) Sodium (Na+) ion (b) Potassium (K+) ion (c) Sodium channel (d) Potassium channel (e) Sodium-Potassium Pump.
Proceduer
- Lidocaine is applied to a patient’s skin before a minor procedure to enable local numbing. Think of a sensory neuron that is activated to transmit pain information to the brain from this area. If the nurse cuts into the skin without adding the Lidocaine, then the neuron will be activated as shown in Figure 12.7 and above. It will generate an action potential that will be transmitted down the neuron. What will happen to the steps 1 – 4 shown in the figure above if Lidocaine is used to numb the patient? Draw steps 1-4, as well as the action potential graph shown in the middle to display the changes expected by applying Lidocaine.
- What will happen after a few hours in which Lidocaine diffuses out of the area? Will the neuron go back to normal action potential generation as shown in Figure 12.7? Explain.
- If you have a patient who ate pufferfish containing Tetrodotoxin, what do you expect will happen to each of the patient’s exposed neurons? Will each neuron be able to generate an action potential? For Figure 12.7 now draw what will happen to steps 1 – 4 and the action potential graph in the presence of Tetrodotoxin.
- Can Tetrodotoxin be used as a local numbing agent like Lidocaine is? Explain.
Exercise 4 Predict the effect of manipulating the neurotransmitter dopamine on addiction
Required Materials
- Scenario: We will go back to Figure 12.1 to revisit the issue of drug abuse or addiction. After having gone through the chapter, you now know that dopamine is a neurotransmitter released as a chemical in response to an action potential in the presynaptic neuron. Once it is released, dopamine binds receptors on the postsynaptic neuron’s cell membrane to generate excitatory PSPs or inhibitory PSPs. Whether the effect is an ESPS or ISPS depends on the type of dopamine receptor on the postsynaptic cell membrane. In this exercise you will explore this synaptic pathway comparing it in normal and addicted brains. You will also think of creative ways in which you can develop medication to treat drug addiction. How can you normalize the dopamine pathways in an addict’s brain?
- Model of dopamine in drug addiction: We will use the Figure 12.1 model showing the changes in dopamine at the synapse that occur in drug addiction.
Procedure
- On the figure above, label the presynaptic and postsynaptic cells and the synaptic cleft.
- In response to cocaine, there is clearly much more dopamine (orange dots) in the synaptic cleft. Using the figure, explain what caused this increase in dopamine level in response to cocaine (compared to what happens in the normal case in response to food). Which process did cocaine block?
- As shown in response to cocaine, the issue that starts addiction has to do with the fact that dopamine persists and continues to signal to the postsynaptic cell highly activating the pleasure or reward centers of the brain for extended periods of time.
If you could develop a chemical treatment to prevent this from occurring, how would you go about it? In other words, what are some ways you can prevent dopamine from accumulating in the presence of cocaine?
Post-laboratory Questions
1. In Exercise 1 you built a 3D model or 3D models of a multipolar neuron. Please answer the following questions related to this exercise.
a. Take a picture of the fully labeled model(s) and paste the picture(s) below.
b. As you built this model, what were some things that you weren’t sure of regarding the model? Did you have questions about the ratio of the cell body to the length of the axon for example? Do you think this would be the same for all multipolar neurons? Discuss.
c. Multipolar neurons are motor neurons that transmit information from the central nervous system to skeletal muscles. Think of all the skeletal muscles in your body that you move on purpose. Think of their distance from the brain’s motor centers. Would you expect the length of the axon of a motor neuron controlling your eyelid muscles to be the same as one controlling you foot muscles? Explain. Use drawing to help answer your question.
d. Did you have any difficulty deciding how wide the diameter of the axon needed to be? Do you think all multipolar neurons have the same diameter or do you expect some to be wider and some narrower? If some neuronal axons are wider, would that help move the action potential down the length of it? Explain.
2. In Exercise 2, you sketched and identified neural tissue components by spinal cord section microscopy. Using the same concepts related to the content of gray matter and white matter and your knowledge of neurons and glial cells, answer the following questions related to another spinal cord section, this time stained with silver stain rather than the Nissl stain used in the slides you observed.
a. Label the following on the low magnification micrograph of the spinal cord below: gray matter, white matter, anterior horns, posterior horns.
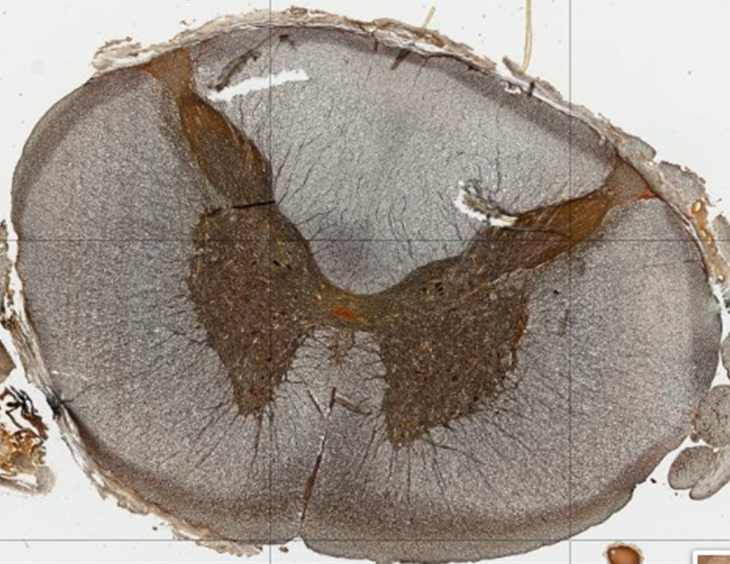
b. In the high magnification image of the same silver stained spinal cord section as above, find and label the following: neuronal cell body, glial cell, myelinated axon.
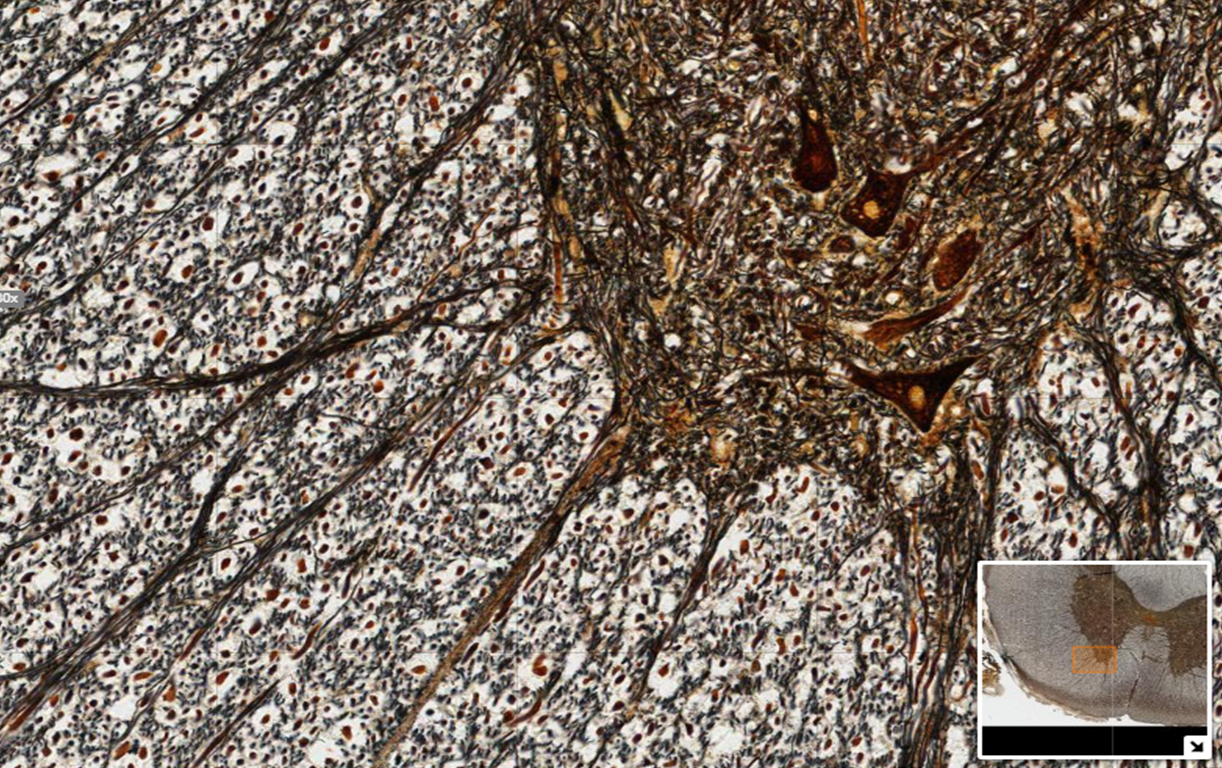
3. In Exercise 3, you explored the effects on action potential of changes to voltage-gated Na+ channels by the local numbing agent Lidocaine and the neurotoxin Tetrodotoxin. Using the same logic and applying your knowledge of how action potentials are generated as shown in Figure 12.7 answer the following questions related to blocking the voltage-gated potassium K+ channel in a neuron.
You are told that your patient has been stung by a scorpion and the scorpion venom contains a potassium K+ channel blocker. There are four steps in the action potential model below. Explain which of these four steps will be affected by blocking the potassium channel and what the effect will be.
- (1) Stimulus to reach threshold?
- (2) Depolarization?
- (3) Repolarization?
- (4) Hyperpolarization?
4. In Exercise 4 you examined ways of manipulating the neurotransmitter dopamine to reduce its effects on addiction. You did this because you were shown that drugs of abuse like cocaine result in an increase in the amount of dopamine therefore heightening the sensation of pleasure for sustained periods of time.
In some patients who suffer from depression, the opposite situation exists: The levels of dopamine becomes too low and therefore the patients do not feel pleasure or get feelings of enjoyment when they should.
a. Draw the synapses comparing the normal reaction to food with the reaction of a depressed person to food (similar to Figure 12.1) showing the levels of dopamine in each.
b. One of the medications that is used to treat depression is a dopamine re-uptake inhibitor. Re-uptake refers to what the dopamine transporters in the figure above do. Explain how a re-uptake inhibitor of dopamine helps a depressed person feel better.
c. Given what you know about the effect of cocaine (and other drugs of abuse) on dopamine and its relation to depression, how do you explain the term “self-medicating” as it refers to use of drugs of abuse and depression?